Notes on Dirac Field
Total Page:16
File Type:pdf, Size:1020Kb
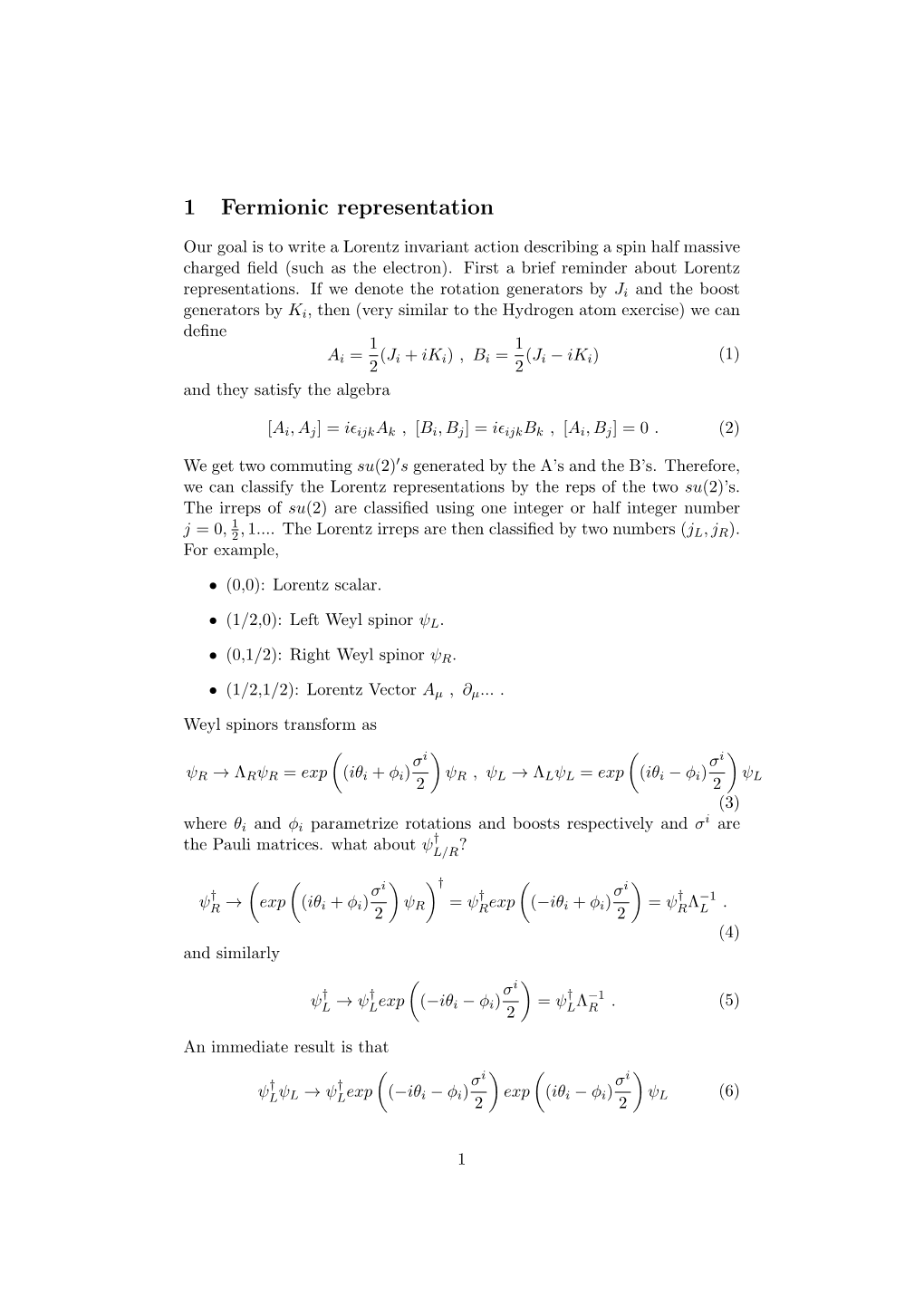
Load more
Recommended publications
-
Effective Dirac Equations in Honeycomb Structures
Effective Dirac equations in honeycomb structures Effective Dirac equations in honeycomb structures Young Researchers Seminar, CERMICS, Ecole des Ponts ParisTech William Borrelli CEREMADE, Universit´eParis Dauphine 11 April 2018 It is self-adjoint on L2(R2; C2) and the spectrum is given by σ(D0) = R; σ(D) = (−∞; −m] [ [m; +1) The domain of the operator and form domain are H1(R2; C2) and 1 2 2 H 2 (R ; C ), respectively. Remark The negative spectrum is associated with antiparticles, in relativistic theories. Effective Dirac equations in honeycomb structures Dirac in 2D The 2D Dirac operator The 2D Dirac operator is defined as D = D0 +mσ3 = −i(σ1@1 + σ2@2) + mσ3: (1) where σk are the Pauli matrices and m ≥ 0 is the mass of the particle. It acts on C2-valued spinors. The domain of the operator and form domain are H1(R2; C2) and 1 2 2 H 2 (R ; C ), respectively. Remark The negative spectrum is associated with antiparticles, in relativistic theories. Effective Dirac equations in honeycomb structures Dirac in 2D The 2D Dirac operator The 2D Dirac operator is defined as D = D0 +mσ3 = −i(σ1@1 + σ2@2) + mσ3: (1) where σk are the Pauli matrices and m ≥ 0 is the mass of the particle. It acts on C2-valued spinors. It is self-adjoint on L2(R2; C2) and the spectrum is given by σ(D0) = R; σ(D) = (−∞; −m] [ [m; +1) Effective Dirac equations in honeycomb structures Dirac in 2D The 2D Dirac operator The 2D Dirac operator is defined as D = D0 +mσ3 = −i(σ1@1 + σ2@2) + mσ3: (1) where σk are the Pauli matrices and m ≥ 0 is the mass of the particle. -
Introductory Lectures on Quantum Field Theory
Introductory Lectures on Quantum Field Theory a b L. Álvarez-Gaumé ∗ and M.A. Vázquez-Mozo † a CERN, Geneva, Switzerland b Universidad de Salamanca, Salamanca, Spain Abstract In these lectures we present a few topics in quantum field theory in detail. Some of them are conceptual and some more practical. They have been se- lected because they appear frequently in current applications to particle physics and string theory. 1 Introduction These notes are based on lectures delivered by L.A.-G. at the 3rd CERN–Latin-American School of High- Energy Physics, Malargüe, Argentina, 27 February–12 March 2005, at the 5th CERN–Latin-American School of High-Energy Physics, Medellín, Colombia, 15–28 March 2009, and at the 6th CERN–Latin- American School of High-Energy Physics, Natal, Brazil, 23 March–5 April 2011. The audience on all three occasions was composed to a large extent of students in experimental high-energy physics with an important minority of theorists. In nearly ten hours it is quite difficult to give a reasonable introduction to a subject as vast as quantum field theory. For this reason the lectures were intended to provide a review of those parts of the subject to be used later by other lecturers. Although a cursory acquaintance with the subject of quantum field theory is helpful, the only requirement to follow the lectures is a working knowledge of quantum mechanics and special relativity. The guiding principle in choosing the topics presented (apart from serving as introductions to later courses) was to present some basic aspects of the theory that present conceptual subtleties. -
5 the Dirac Equation and Spinors
5 The Dirac Equation and Spinors In this section we develop the appropriate wavefunctions for fundamental fermions and bosons. 5.1 Notation Review The three dimension differential operator is : ∂ ∂ ∂ = , , (5.1) ∂x ∂y ∂z We can generalise this to four dimensions ∂µ: 1 ∂ ∂ ∂ ∂ ∂ = , , , (5.2) µ c ∂t ∂x ∂y ∂z 5.2 The Schr¨odinger Equation First consider a classical non-relativistic particle of mass m in a potential U. The energy-momentum relationship is: p2 E = + U (5.3) 2m we can substitute the differential operators: ∂ Eˆ i pˆ i (5.4) → ∂t →− to obtain the non-relativistic Schr¨odinger Equation (with = 1): ∂ψ 1 i = 2 + U ψ (5.5) ∂t −2m For U = 0, the free particle solutions are: iEt ψ(x, t) e− ψ(x) (5.6) ∝ and the probability density ρ and current j are given by: 2 i ρ = ψ(x) j = ψ∗ ψ ψ ψ∗ (5.7) | | −2m − with conservation of probability giving the continuity equation: ∂ρ + j =0, (5.8) ∂t · Or in Covariant notation: µ µ ∂µj = 0 with j =(ρ,j) (5.9) The Schr¨odinger equation is 1st order in ∂/∂t but second order in ∂/∂x. However, as we are going to be dealing with relativistic particles, space and time should be treated equally. 25 5.3 The Klein-Gordon Equation For a relativistic particle the energy-momentum relationship is: p p = p pµ = E2 p 2 = m2 (5.10) · µ − | | Substituting the equation (5.4), leads to the relativistic Klein-Gordon equation: ∂2 + 2 ψ = m2ψ (5.11) −∂t2 The free particle solutions are plane waves: ip x i(Et p x) ψ e− · = e− − · (5.12) ∝ The Klein-Gordon equation successfully describes spin 0 particles in relativistic quan- tum field theory. -
WEYL SPINORS and DIRAC's ELECTRON EQUATION C William O
WEYL SPINORS AND DIRAC’SELECTRON EQUATION c William O. Straub, PhD Pasadena, California March 17, 2005 I’ve been planning for some time now to provide a simplified write-up of Weyl’s seminal 1929 paper on gauge invariance. I’m still planning to do it, but since Weyl’s paper covers so much ground I thought I would first address a discovery that he made kind of in passing that (as far as I know) has nothing to do with gauge-invariant gravitation. It involves the mathematical objects known as spinors. Although Weyl did not invent spinors, I believe he was the first to explore them in the context of Dirac’srelativistic electron equation. However, without a doubt Weyl was the first person to investigate the consequences of zero mass in the Dirac equation and the implications this has on parity conservation. In doing so, Weyl unwittingly anticipated the existence of a particle that does not respect the preservation of parity, an unheard-of idea back in 1929 when parity conservation was a sacred cow. Following that, I will use this opportunity to derive the Dirac equation itself and talk a little about its role in particle spin. Those of you who have studied Dirac’s relativistic electron equation may know that the 4-component Dirac spinor is actually composed of two 2-component spinors that Weyl introduced to physics back in 1929. The Weyl spinors have unusual parity properties, and because of this Pauli was initially very critical of Weyl’sanalysis because it postulated massless fermions (neutrinos) that violated the then-cherished notion of parity conservation. -
2 Lecture 1: Spinors, Their Properties and Spinor Prodcuts
2 Lecture 1: spinors, their properties and spinor prodcuts Consider a theory of a single massless Dirac fermion . The Lagrangian is = ¯ i@ˆ . (2.1) L ⇣ ⌘ The Dirac equation is i@ˆ =0, (2.2) which, in momentum space becomes pUˆ (p)=0, pVˆ (p)=0, (2.3) depending on whether we take positive-energy(particle) or negative-energy (anti-particle) solutions of the Dirac equation. Therefore, in the massless case no di↵erence appears in equations for paprticles and anti-particles. Finding one solution is therefore sufficient. The algebra is simplified if we take γ matrices in Weyl repreentation where µ µ 0 σ γ = µ . (2.4) " σ¯ 0 # and σµ =(1,~σ) andσ ¯µ =(1, ~ ). The Pauli matrices are − 01 0 i 10 σ = ,σ= − ,σ= . (2.5) 1 10 2 i 0 3 0 1 " # " # " − # The matrix γ5 is taken to be 10 γ5 = − . (2.6) " 01# We can use the matrix γ5 to construct projection operators on to upper and lower parts of the four-component spinors U and V . The projection operators are 1 γ 1+γ Pˆ = − 5 , Pˆ = 5 . (2.7) L 2 R 2 Let us write u (p) U(p)= L , (2.8) uR(p) ! where uL(p) and uR(p) are two-component spinors. Since µ 0 pµσ pˆ = µ , (2.9) " pµσ¯ 0(p) # andpU ˆ (p) = 0, the two-component spinors satisfy the following (Weyl) equations µ µ pµσ uR(p)=0,pµσ¯ uL(p)=0. (2.10) –3– Suppose that we have a left handed spinor uL(p) that satisfies the Weyl equation. -
The Bound States of Dirac Equation with a Scalar Potential
THE BOUND STATES OF DIRAC EQUATION WITH A SCALAR POTENTIAL BY VATSAL DWIVEDI THESIS Submitted in partial fulfillment of the requirements for the degree of Master of Science in Applied Mathematics in the Graduate College of the University of Illinois at Urbana-Champaign, 2015 Urbana, Illinois Adviser: Professor Jared Bronski Abstract We study the bound states of the 1 + 1 dimensional Dirac equation with a scalar potential, which can also be interpreted as a position dependent \mass", analytically as well as numerically. We derive a Pr¨ufer-like representation for the Dirac equation, which can be used to derive a condition for the existence of bound states in terms of the fixed point of the nonlinear Pr¨uferequation for the angle variable. Another condition was derived by interpreting the Dirac equation as a Hamiltonian flow on R4 and a shooting argument for the induced flow on the space of Lagrangian planes of R4, following a similar calculation by Jones (Ergodic Theor Dyn Syst, 8 (1988) 119-138). The two conditions are shown to be equivalent, and used to compute the bound states analytically and numerically, as well as to derive a Calogero-like upper bound on the number of bound states. The analytic computations are also compared to the bound states computed using techniques from supersymmetric quantum mechanics. ii Acknowledgments In the eternity that is the grad school, this project has been what one might call an impulsive endeavor. In the 6 months from its inception to its conclusion, it has, without doubt, greatly benefited from quite a few individuals, as well as entities, around me, to whom I owe my sincere regards and gratitude. -
Introduction to Supersymmetry
Introduction to Supersymmetry Pre-SUSY Summer School Corpus Christi, Texas May 15-18, 2019 Stephen P. Martin Northern Illinois University [email protected] 1 Topics: Why: Motivation for supersymmetry (SUSY) • What: SUSY Lagrangians, SUSY breaking and the Minimal • Supersymmetric Standard Model, superpartner decays Who: Sorry, not covered. • For some more details and a slightly better attempt at proper referencing: A supersymmetry primer, hep-ph/9709356, version 7, January 2016 • TASI 2011 lectures notes: two-component fermion notation and • supersymmetry, arXiv:1205.4076. If you find corrections, please do let me know! 2 Lecture 1: Motivation and Introduction to Supersymmetry Motivation: The Hierarchy Problem • Supermultiplets • Particle content of the Minimal Supersymmetric Standard Model • (MSSM) Need for “soft” breaking of supersymmetry • The Wess-Zumino Model • 3 People have cited many reasons why extensions of the Standard Model might involve supersymmetry (SUSY). Some of them are: A possible cold dark matter particle • A light Higgs boson, M = 125 GeV • h Unification of gauge couplings • Mathematical elegance, beauty • ⋆ “What does that even mean? No such thing!” – Some modern pundits ⋆ “We beg to differ.” – Einstein, Dirac, . However, for me, the single compelling reason is: The Hierarchy Problem • 4 An analogy: Coulomb self-energy correction to the electron’s mass A point-like electron would have an infinite classical electrostatic energy. Instead, suppose the electron is a solid sphere of uniform charge density and radius R. An undergraduate problem gives: 3e2 ∆ECoulomb = 20πǫ0R 2 Interpreting this as a correction ∆me = ∆ECoulomb/c to the electron mass: 15 0.86 10− meters m = m + (1 MeV/c2) × . -
Relativistic Quantum Mechanics 1
Relativistic Quantum Mechanics 1 The aim of this chapter is to introduce a relativistic formalism which can be used to describe particles and their interactions. The emphasis 1.1 SpecialRelativity 1 is given to those elements of the formalism which can be carried on 1.2 One-particle states 7 to Relativistic Quantum Fields (RQF), which underpins the theoretical 1.3 The Klein–Gordon equation 9 framework of high energy particle physics. We begin with a brief summary of special relativity, concentrating on 1.4 The Diracequation 14 4-vectors and spinors. One-particle states and their Lorentz transforma- 1.5 Gaugesymmetry 30 tions follow, leading to the Klein–Gordon and the Dirac equations for Chaptersummary 36 probability amplitudes; i.e. Relativistic Quantum Mechanics (RQM). Readers who want to get to RQM quickly, without studying its foun- dation in special relativity can skip the first sections and start reading from the section 1.3. Intrinsic problems of RQM are discussed and a region of applicability of RQM is defined. Free particle wave functions are constructed and particle interactions are described using their probability currents. A gauge symmetry is introduced to derive a particle interaction with a classical gauge field. 1.1 Special Relativity Einstein’s special relativity is a necessary and fundamental part of any Albert Einstein 1879 - 1955 formalism of particle physics. We begin with its brief summary. For a full account, refer to specialized books, for example (1) or (2). The- ory oriented students with good mathematical background might want to consult books on groups and their representations, for example (3), followed by introductory books on RQM/RQF, for example (4). -
Charge Conjugation Symmetry
Charge Conjugation Symmetry In the previous set of notes we followed Dirac's original construction of positrons as holes in the electron's Dirac sea. But the modern point of view is rather different: The Dirac sea is experimentally undetectable | it's simply one of the aspects of the physical ? vacuum state | and the electrons and the positrons are simply two related particle species. Moreover, the electrons and the positrons have exactly the same mass but opposite electric charges. Many other particle species exist in similar particle-antiparticle pairs. The particle and the corresponding antiparticle have exactly the same mass but opposite electric charges, as well as other conserved charges such as the lepton number or the baryon number. Moreover, the strong and the electromagnetic interactions | but not the weak interactions | respect the change conjugation symmetry which turns particles into antiparticles and vice verse, C^ jparticle(p; s)i = jantiparticle(p; s)i ; C^ jantiparticle(p; s)i = jparticle(p; s)i ; (1) − + + − for example C^ e (p; s) = e (p; s) and C^ e (p; s) = e (p; s) . In light of this sym- metry, deciding which particle species is particle and which is antiparticle is a matter of convention. For example, we know that the charged pions π+ and π− are each other's an- tiparticles, but it's up to our choice whether we call the π+ mesons particles and the π− mesons antiparticles or the other way around. In the Hilbert space of the quantum field theory, the charge conjugation operator C^ is a unitary operator which squares to 1, thus C^ 2 = 1 =) C^ y = C^ −1 = C^:; (2) ? In condensed matter | say, in a piece of semiconductor | we may detect the filled electron states by making them interact with the outside world. -
7 Quantized Free Dirac Fields
7 Quantized Free Dirac Fields 7.1 The Dirac Equation and Quantum Field Theory The Dirac equation is a relativistic wave equation which describes the quantum dynamics of spinors. We will see in this section that a consistent description of this theory cannot be done outside the framework of (local) relativistic Quantum Field Theory. The Dirac Equation (i∂/ m)ψ =0 ψ¯(i∂/ + m) = 0 (1) − can be regarded as the equations of motion of a complex field ψ. Much as in the case of the scalar field, and also in close analogy to the theory of non-relativistic many particle systems discussed in the last chapter, the Dirac field is an operator which acts on a Fock space. We have already discussed that the Dirac equation also follows from a least-action-principle. Indeed the Lagrangian i µ = [ψ¯∂ψ/ (∂µψ¯)γ ψ] mψψ¯ ψ¯(i∂/ m)ψ (2) L 2 − − ≡ − has the Dirac equation for its equation of motion. Also, the momentum Πα(x) canonically conjugate to ψα(x) is ψ δ † Πα(x)= L = iψα (3) δ∂0ψα(x) Thus, they obey the equal-time Poisson Brackets ψ 3 ψα(~x), Π (~y) P B = iδαβδ (~x ~y) (4) { β } − Thus † 3 ψα(~x), ψ (~y) P B = δαβδ (~x ~y) (5) { β } − † In other words the field ψα and its adjoint ψα are a canonical pair. This result follows from the fact that the Dirac Lagrangian is first order in time derivatives. Notice that the field theory of non-relativistic many-particle systems (for both fermions on bosons) also has a Lagrangian which is first order in time derivatives. -
Spin-1/2 Fermions in Quantum Field Theory
Spin-1/2 fermions in quantum field theory µ First, recall that 4-vectors transform under Lorentz transformations, Λ ν, as p′ µ = Λµ pν, where Λ SO(3,1) satisfies Λµ g Λρ = g .∗ A Lorentz ν ∈ ν µρ λ νλ transformation corresponds to a rotation by θ about an axis nˆ [θ~ θnˆ] and ≡ a boost, ζ~ = vˆ tanh−1 ~v , where ~v is the corresponding velocity. Under the | | same Lorentz transformation, a generic field transforms as: ′ ′ Φ (x )= MR(Λ)Φ(x) , where M exp iθ~·J~ iζ~·K~ are N N representation matrices of R ≡ − − × 1 1 the Lorentz group. Defining J~+ (J~ + iK~ ) and J~− (J~ iK~ ), ≡ 2 ≡ 2 − i j ijk k i j [J± , J±]= iǫ J± , [J± , J∓] = 0 . Thus, the representations of the Lorentz algebra are characterized by (j1,j2), 1 1 where the ji are half-integers. (0, 0) is a scalar and (2, 2) is a four-vector. Of 1 1 interest to us here are the spinor representations (2, 0) and (0, 2). ∗ In our conventions, gµν = diag(1 , −1 , −1 , −1). (1, 0): M = exp i θ~·~σ 1ζ~·~σ , butalso (M −1)T = iσ2M(iσ2)−1 2 −2 − 2 (0, 1): [M −1]† = exp i θ~·~σ + 1ζ~·~σ , butalso M ∗ = iσ2[M −1]†(iσ2)−1 2 −2 2 since (iσ2)~σ(iσ2)−1 = ~σ∗ = ~σT − − Transformation laws of 2-component fields ′ β ξα = Mα ξβ , ′ α −1 T α β ξ = [(M ) ] β ξ , ′† α˙ −1 † α˙ † β˙ ξ = [(M ) ] β˙ ξ , ˙ ξ′† =[M ∗] βξ† . -
Time Reversal Symmetry
Contents Contents i List of Figures ii List of Tables iii 7 Time Reversal 1 7.1 The Poincar´eGroup ........................................ 1 7.1.1 Space inversion and time-reversal ............................ 1 7.1.2 Representations of the Poincar´eLie algebra ...................... 2 7.1.3 Whither time-reversal? .................................. 3 7.2 Antilinearity : The Solution to All Our Problems ........................ 4 7.2.1 Properties of antilinear operators ............................ 4 7.2.2 Position and momentum eigenstates .......................... 6 7.2.3 Change of basis for time-reversal ............................ 7 7.2.4 Time reversal with spin .................................. 8 7.2.5 Kramers degeneracy ................................... 9 7.2.6 Externalfields ....................................... 9 7.3 Time Reversal and Point Group Symmetries .......................... 10 7.3.1 Complex conjugate representations ........................... 11 7.3.2 Generalized time-reversal ................................ 12 7.4 Consequences of Time-Reversal ................................. 13 i ii CONTENTS 7.4.1 Selection rules and time-reversal ............................ 13 7.4.2 Onsager reciprocity .................................... 14 7.5 Colorgroups ............................................ 16 7.5.1 Magnetic Bravais lattices and magnetic space groups ................. 19 7.5.2 Corepresentations of color groups ............................ 23 7.6 Appendix : The Foldy-Wouthuysen Transformation .....................