Early Mississippian Ocean Anoxia Triggering
Total Page:16
File Type:pdf, Size:1020Kb
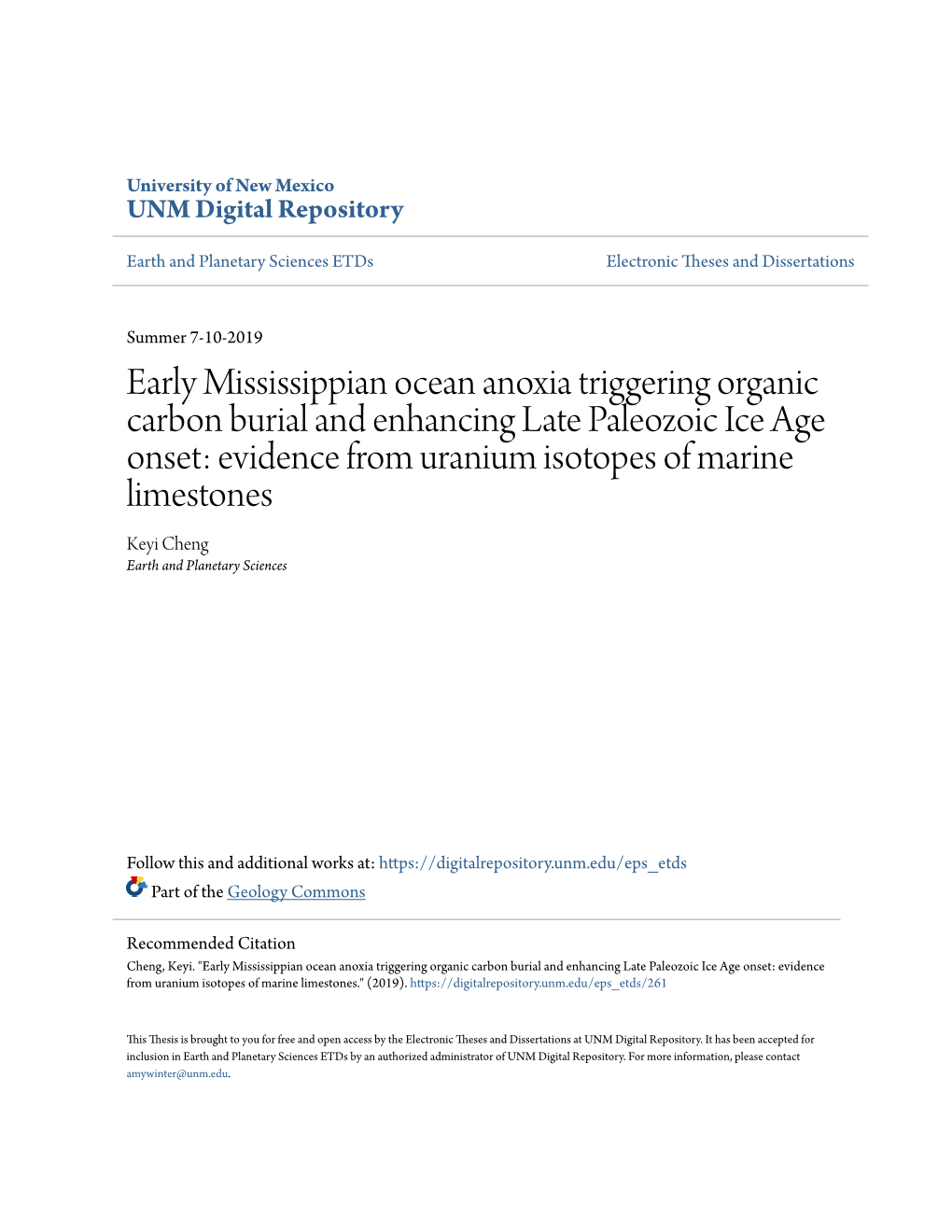
Load more
Recommended publications
-
Chapter 2 Paleozoic Stratigraphy of the Grand Canyon
CHAPTER 2 PALEOZOIC STRATIGRAPHY OF THE GRAND CANYON PAIGE KERCHER INTRODUCTION The Paleozoic Era of the Phanerozoic Eon is defined as the time between 542 and 251 million years before the present (ICS 2010). The Paleozoic Era began with the evolution of most major animal phyla present today, sparked by the novel adaptation of skeletal hard parts. Organisms continued to diversify throughout the Paleozoic into increasingly adaptive and complex life forms, including the first vertebrates, terrestrial plants and animals, forests and seed plants, reptiles, and flying insects. Vast coal swamps covered much of mid- to low-latitude continental environments in the late Paleozoic as the supercontinent Pangaea began to amalgamate. The hardiest taxa survived the multiple global glaciations and mass extinctions that have come to define major time boundaries of this era. Paleozoic North America existed primarily at mid to low latitudes and experienced multiple major orogenies and continental collisions. For much of the Paleozoic, North America’s southwestern margin ran through Nevada and Arizona – California did not yet exist (Appendix B). The flat-lying Paleozoic rocks of the Grand Canyon, though incomplete, form a record of a continental margin repeatedly inundated and vacated by shallow seas (Appendix A). IMPORTANT STRATIGRAPHIC PRINCIPLES AND CONCEPTS • Principle of Original Horizontality – In most cases, depositional processes produce flat-lying sedimentary layers. Notable exceptions include blanketing ash sheets, and cross-stratification developed on sloped surfaces. • Principle of Superposition – In an undisturbed sequence, older strata lie below younger strata; a package of sedimentary layers youngs upward. • Principle of Lateral Continuity – A layer of sediment extends laterally in all directions until it naturally pinches out or abuts the walls of its confining basin. -
Speleothem Paleoclimatology for the Caribbean, Central America, and North America
quaternary Review Speleothem Paleoclimatology for the Caribbean, Central America, and North America Jessica L. Oster 1,* , Sophie F. Warken 2,3 , Natasha Sekhon 4, Monica M. Arienzo 5 and Matthew Lachniet 6 1 Department of Earth and Environmental Sciences, Vanderbilt University, Nashville, TN 37240, USA 2 Department of Geosciences, University of Heidelberg, 69120 Heidelberg, Germany; [email protected] 3 Institute of Environmental Physics, University of Heidelberg, 69120 Heidelberg, Germany 4 Department of Geological Sciences, Jackson School of Geosciences, University of Texas, Austin, TX 78712, USA; [email protected] 5 Desert Research Institute, Reno, NV 89512, USA; [email protected] 6 Department of Geoscience, University of Nevada, Las Vegas, NV 89154, USA; [email protected] * Correspondence: [email protected] Received: 27 December 2018; Accepted: 21 January 2019; Published: 28 January 2019 Abstract: Speleothem oxygen isotope records from the Caribbean, Central, and North America reveal climatic controls that include orbital variation, deglacial forcing related to ocean circulation and ice sheet retreat, and the influence of local and remote sea surface temperature variations. Here, we review these records and the global climate teleconnections they suggest following the recent publication of the Speleothem Isotopes Synthesis and Analysis (SISAL) database. We find that low-latitude records generally reflect changes in precipitation, whereas higher latitude records are sensitive to temperature and moisture source variability. Tropical records suggest precipitation variability is forced by orbital precession and North Atlantic Ocean circulation driven changes in atmospheric convection on long timescales, and tropical sea surface temperature variations on short timescales. On millennial timescales, precipitation seasonality in southwestern North America is related to North Atlantic climate variability. -
Geochemical Characteristics of Late Ordovician Shales in the Upper Yangtze Platform, South China: Implications for Redox Environmental Evolution
minerals Article Geochemical Characteristics of Late Ordovician Shales in the Upper Yangtze Platform, South China: Implications for Redox Environmental Evolution Donglin Lin 1,2,3, Shuheng Tang 1,2,3,*, Zhaodong Xi 1,2,3, Bing Zhang 1,2,3 and Yapei Ye 1,2,3 1 School of Energy Resource, China University of Geosciences, Beijing 100083, China; [email protected] (D.L.); [email protected] (Z.X.); [email protected] (B.Z.); [email protected] (Y.Y.) 2 Key Laboratory of Marine Reservoir Evolution and Hydrocarbon Enrichment Mechanism, Ministry of Education, Beijing 100083, China 3 Key Laboratory of Strategy Evaluation for Shale Gas, Ministry of Land and Resources, Beijing 100083, China * Correspondence: [email protected]; Tel.: +86-134-8882-1576 Abstract: Changes to the redox environment of seawater in the Late Ordovician affect the process of organic matter enrichment and biological evolution. However, the evolution of redox and its underlying causes remain unclear. This paper analyzed the vertical variability of main, trace elements and δ34Spy from a drill core section (well ZY5) in the Upper Yangtze Platform, and described the redox conditions, paleoproductivity and paleoclimate variability recorded in shale deposits of the P. pacificus zone and M. extraordinarius zone that accumulated during Wufeng Formation. The results showed that shale from well ZY5 in Late Ordovician was deposited under oxidized water environment, and Citation: Lin, D.; Tang, S.; Xi, Z.; Zhang, B.; Ye, Y. Geochemical there are more strongly reducing bottom water conditions of the M. extraordinarius zone compared Characteristics of Late Ordovician with the P. -
Pennsylvanian Boundary Unconformity in Marine Carbonate Successions
University of Nebraska - Lincoln DigitalCommons@University of Nebraska - Lincoln Dissertations & Theses in Earth and Atmospheric Earth and Atmospheric Sciences, Department of Sciences Summer 6-2014 ORIGIN AND DISTRIBUTION OF THE MISSISSIPPIAN – PENNSYLVANIAN BOUNDARY UNCONFORMITY IN MARINE CARBONATE SUCCESSIONS WITH A CASE STUDY OF THE KARST DEVELOPMENT ATOP THE MADISON FORMATION IN THE BIGHORN BASIN, WYOMING. Lucien Nana Yobo University of Nebraska-Lincoln, [email protected] Follow this and additional works at: http://digitalcommons.unl.edu/geoscidiss Part of the Geochemistry Commons, Geology Commons, Sedimentology Commons, and the Stratigraphy Commons Nana Yobo, Lucien, "ORIGIN AND DISTRIBUTION OF THE MISSISSIPPIAN – PENNSYLVANIAN BOUNDARY UNCONFORMITY IN MARINE CARBONATE SUCCESSIONS WITH A CASE STUDY OF THE KARST DEVELOPMENT ATOP THE MADISON FORMATION IN THE BIGHORN BASIN, WYOMING." (2014). Dissertations & Theses in Earth and Atmospheric Sciences. 59. http://digitalcommons.unl.edu/geoscidiss/59 This Article is brought to you for free and open access by the Earth and Atmospheric Sciences, Department of at DigitalCommons@University of Nebraska - Lincoln. It has been accepted for inclusion in Dissertations & Theses in Earth and Atmospheric Sciences by an authorized administrator of DigitalCommons@University of Nebraska - Lincoln. ORIGIN AND DISTRIBUTION OF THE MISSISSIPPIAN – PENNSYLVANIAN BOUNDARY UNCONFORMITY IN MARINE CARBONATE SUCCESSIONS WITH A CASE STUDY OF THE KARST DEVELOPMENT ATOP THE MADISON FORMATION IN THE BIGHORN BASIN, WYOMING. By Luscalors Lucien Nana Yobo A THESIS Presented to the Faculty of The Graduate College at the University of Nebraska In Partial Fulfillment of Requirements For the Degree of Master of Science Major: Earth and Atmospheric Sciences Under the Supervision of Professor Tracy D. -
Geologic Timeline
SCIENCE IN THE PARK: GEOLOGY GEOLOGIC TIME SCALE ANALOGY PURPOSE: To show students the order of events and time periods in geologic time and the order of events and ages of the physiographic provinces in Virginia. BACKGROUND: Exact dates for events change as scientists explore geologic time. Dates vary from resource to resource and may not be the same as the dates that appear in your text book. Analogies for geologic time: a 24 hour clock or a yearly calendar. Have students or groups of students come up with their own original analogy. Before you assign this activity, you may want to try it, depending on the age of the student, level of the class, or time constraints, you may want to leave out the events that have a date of less than 1 million years. ! Review conversions in the metric system before you begin this activity ! References L.S. Fichter, 1991 (1997) http://csmres.jmu.edu/geollab/vageol/vahist/images/Vahistry.PDF http://pubs.usgs.gov/gip/geotime/age.html Wicander, Reed. Historical Geology. Fourth Edition. Toronto, Ontario: Brooks/Cole, 2004. Print. VIRGINIA STANDARDS OF LEARNING ES.10 The student will investigate and understand that many aspects of the history and evolution of the Earth can be inferred by studying rocks and fossils. Key concepts include: relative and absolute dating; rocks and fossils from many different geologic periods and epochs are found in Virginia. Developed by C.P. Anderson Page 1 SCIENCE IN THE PARK: GEOLOGY Building a Geologic Time Scale Time: Materials Meter stick, 5 cm adding machine tape, pencil, colored pencils Procedure 1. -
Jocelyn A. Sessa
Jocelyn A. Sessa Current: Assistant Curator of Invertebrate Paleontology, Academy of Natural Sciences, & Assistant Professor, Department of Biodiversity, Earth & Environmental Science of Drexel University. Past Positions: 2016 to 2017 Senior Scientist in Paleontology & Education, American Museum of Natural History. Postdoctoral Fellowships: 2012 to 2016 Departments of Paleontology & Education, American Museum of Natural History. 2010 to 2012 Department of Paleobiology, Smithsonian National Museum of Natural History. 2009 to 2010 Department of Earth Sciences, Syracuse University. Education: Ph.D., 2009 Department of Geosciences, Pennsylvania State University, University Park, PA. M.S., 2003 Department of Geology, University of Cincinnati, Cincinnati, Ohio. B.A., 2000 Department of Geological Sciences, State University of New York at Geneseo, Geneseo, NY. Cum laude, minor in Environmental Studies. Publications (* indicates student author; for student work, ‡ indicates corresponding author): Buczek, A.J.*, Hendy, A., Hopkins, M. Sessa, J.A.‡ 2020. On the reconciliation of biostratigraphy and strontium isotope stratigraphy of three southern Californian Plio-Pleistocene formations. Geological Society of America Bulletin 132 ; doi.org/10.1130/B35488.1. Oakes, R.L., Sessa, J.A. 2020. Determining how biotic and abiotic variables affect the shell condition and parameters of Heliconoides inflatus pteropods from a sediment trap in the Cariaco Basin. Biogeosciences 17:1975–1990; doi.org/10.5194/bg-17-1975-2020. Oakes, R.L., Hill Chase, M., Siddall, M.E., Sessa, J.A. 2020. Testing the impact of two key scan parameters on the quality and repeatability of measurements from CT scan data. Palaeontologia Electronica 23(1):a07; doi.org/10.26879/942. Ferguson, K.*, MacLeod, K.G.‡, Landman, N.H., Sessa, J.A.‡ 2019. -
Tournaisian and Viséan Lophophyllum of Gorskiy (1932)
Geologos 23, 3 (2017): 215–221 doi: 10.1515/logos-2017-0022 Tournaisian and Viséan Lophophyllum of Gorskiy (1932) from the Kirghiz Steppe and a possible ancestor of a new Bashkirian rugose coral genus from the Donets Basin (Ukraine) Jerzy Fedorowski Institute of Geology, Adam Mickiewicz University, Bogumiła Krygowskiego 12, 61-680 Poznań, Poland; e-mail: [email protected] Abstract All specimens assigned by Gorskiy (1932) to the genus Lophophyllum Milne Edwards and Haime, 1850 are revised, rede- scribed and reillustrated. The corallite identified by him as a second, specifically indeterminate species ofLophophyllum is here questionably included in Amygdalophyllum Dun and Benson, 1920. For the reminding specimens two new, un- named genera are suggested. ”Lophophyllum” subtortuosum Gorskiy, 1932 belongs to a new, non-dissepimented genus of an unknown family. A possible relationship between gen. nov. 1, sp. nov. 1 and the new Bashkirian genus from the Donets Basin (Ukraine) is proposed. Key words: Kyrgyzstan, “Lophophyllum”, Rugosa, Lower Carboniferous, revision 1. Introduction applied by him cannot be adopted without revision. In addition, his conclusions concerning the relation- The rugose corals redescribed in the present note ships of the Kirghiz Steppe coral fauna cannot be ac- form a small part of the diversified coral fauna cepted without such a revision. The close similarity described by Gorskiy (1932) from the Devonian/ or relationship of the coral fauna from the Kirghiz Carboniferous passage beds and from Tournaisian Steppe to corals from the United States mentioned to lower Viséan strata of the Kirghiz Steppe of the by Gorskiy (1932, p. 58) is particularly doubtful. -
Carboniferous Formations and Faunas of Central Montana
Carboniferous Formations and Faunas of Central Montana GEOLOGICAL SURVEY PROFESSIONAL PAPER 348 Carboniferous Formations and Faunas of Central Montana By W. H. EASTON GEOLOGICAL SURVEY PROFESSIONAL PAPER 348 A study of the stratigraphic and ecologic associa tions and significance offossils from the Big Snowy group of Mississippian and Pennsylvanian rocks UNITED STATES GOVERNMENT PRINTING OFFICE, WASHINGTON : 1962 UNITED STATES DEPARTMENT OF THE INTERIOR STEWART L. UDALL, Secretary GEOLOGICAL SURVEY Thomas B. Nolan, Director The U.S. Geological Survey Library has cataloged this publication as follows : Eastern, William Heyden, 1916- Carboniferous formations and faunas of central Montana. Washington, U.S. Govt. Print. Off., 1961. iv, 126 p. illus., diagrs., tables. 29 cm. (U.S. Geological Survey. Professional paper 348) Part of illustrative matter folded in pocket. Bibliography: p. 101-108. 1. Paleontology Montana. 2. Paleontology Carboniferous. 3. Geology, Stratigraphic Carboniferous. I. Title. (Series) For sale by the Superintendent of Documents, U.S. Government Printing Office Washington 25, B.C. CONTENTS Page Page Abstract-__________________________________________ 1 Faunal analysis Continued Introduction _______________________________________ 1 Faunal relations ______________________________ 22 Purposes of the study_ __________________________ 1 Long-ranging elements...__________________ 22 Organization of present work___ __________________ 3 Elements of Mississippian affinity.._________ 22 Acknowledgments--.-------.- ___________________ -
Tectonic Features of the Precambrian Belt Basin and Their Influence on Post-Belt Structures
... Tectonic Features of the .., Precambrian Belt Basin and Their Influence on Post-Belt Structures GEOLOGICAL SURVEY PROFESSIONAL PAPER 866 · Tectonic Features of the · Precambrian Belt Basin and Their Influence on Post-Belt Structures By JACK E. HARRISON, ALLAN B. GRIGGS, and JOHN D. WELLS GEOLOGICAL SURVEY PROFESSIONAL PAPER X66 U N IT ED STATES G 0 V ERN M EN T P R I NT I N G 0 F F I C E, \VAS H I N G T 0 N 19 7 4 UNITED STATES DEPARTMENT OF THE INTERIOR ROGERS C. B. MORTON, Secretary GEOLOGICAL SURVEY V. E. McKelvey, Director Library of Congress catalog-card No. 74-600111 ) For sale by the Superintendent of Documents, U.S. GO\·ernment Printing Office 'Vashington, D.C. 20402 - Price 65 cents (paper cO\·er) Stock Number 2401-02554 CONTENTS Page Page Abstract................................................. 1 Phanerozoic events-Continued Introduction . 1 Late Mesozoic through early Tertiary-Continued Genesis and filling of the Belt basin . 1 Idaho batholith ................................. 7 Is the Belt basin an aulacogen? . 5 Boulder batholith ............................... 8 Precambrian Z events . 5 Northern Montana disturbed belt ................. 8 Phanerozoic events . 5 Tectonics along the Lewis and Clark line .............. 9 Paleozoic through early Mesozoic . 6 Late Cenozoic block faults ........................... 13 Late Mesozoic through early Tertiary . 6 Conclusions ............................................. 13 Kootenay arc and mobile belt . 6 References cited ......................................... 14 ILLUSTRATIONS Page FIGURES 1-4. Maps: 1. Principal basins of sedimentation along the U.S.-Canadian Cordillera during Precambrian Y time (1,600-800 m.y. ago) ............................................................................................... 2 2. Principal tectonic elements of the Belt basin reentrant as inferred from the sedimentation record ............ -
Climate Change
Optional EffectWhatAddendum: Isof Causing Climate Weather Climate Change and Change,on HumansClimate and and How the DoClimate Environment We Know? Change Optional EffectWhatAddendum: Isof Causing Climate Weather Climate Change and Change,on HumansClimate and and How the DoClimate Environment We Know? Change Part I: The Claim Two students are discussing what has caused the increase in temperature in the last century (100 years). Optional EffectWhatAddendum: Isof Causing Climate Weather Climate Change and Change,on HumansClimate and and How the DoClimate Environment We Know? Change Part II: What Do We Still Need to Know? Destiny Emilio Know from Task 1 Need to Know • Has this happened in • Is more carbon the past? dioxide being made • Are we just in a now than in the past? warm period? • Are humans responsible? Optional EffectWhatAddendum: Isof Causing Climate Weather Climate Change and Change,on HumansClimate and and How the DoClimate Environment We Know? Change Part III: Collect Evidence To support a claim, we need evidence!!! Optional EffectWhatAddendum: Isof Causing Climate Weather Climate Change and Change,on HumansClimate and and How the DoClimate Environment We Know? Change Climate Readings to the Present Data: Petit, J.R., et al., 2001, Vostok Ice Core Data for 420,000 Years, IGBP PAGES/World Data Center for Paleoclimatology Data Contribution Series #2001-076. NOAA/NGDC Paleoclimatology Program, Boulder CO, USA. https://www1.ncdc.noaa.gov/pub/data/paleo/icecore/antarctica/vostok/deutnat.tXt, …/co2nat.tXt Optional EffectWhatAddendum: Isof Causing Climate Weather Climate Change and Change,on HumansClimate and and How the DoClimate Environment We Know? Change How do we know about the climate from thousands of years ago? Optional EffectWhatAddendum: Isof Causing Climate Weather Climate Change and Change,on HumansClimate and and How the DoClimate Environment We Know? Change Measuring Devices • Thermometers were not invented until 1724. -
Microbial Diversity Under Extreme Euxinia: Mahoney Lake, Canada V
Geobiology (2012), 10, 223–235 DOI: 10.1111/j.1472-4669.2012.00317.x Microbial diversity under extreme euxinia: Mahoney Lake, Canada V. KLEPAC-CERAJ,1,2 C. A. HAYES,3 W. P. GILHOOLY,4 T. W. LYONS,5 R. KOLTER2 AND A. PEARSON3 1Department of Molecular Genetics, Forsyth Institute, Cambridge, MA, USA 2Department of Microbiology and Molecular Genetics, Harvard Medical School, Boston, MA, USA 3Department of Earth and Planetary Sciences, Harvard University, Cambridge, MA, USA 4Department of Earth and Planetary Sciences, Washington University, Saint Louis, MO, USA 5Department of Earth Sciences, University of California, Riverside, CA, USA ABSTRACT Mahoney Lake, British Columbia, Canada, is a stratified, 15-m deep saline lake with a euxinic (anoxic, sulfidic) hypolimnion. A dense plate of phototrophic purple sulfur bacteria is found at the chemocline, but to date the rest of the Mahoney Lake microbial ecosystem has been underexamined. In particular, the microbial community that resides in the aphotic hypolimnion and ⁄ or in the lake sediments is unknown, and it is unclear whether the sulfate reducers that supply sulfide for phototrophy live only within, or also below, the plate. Here we profiled distribu- tions of 16S rRNA genes using gene clone libraries and PhyloChip microarrays. Both approaches suggest that microbial diversity is greatest in the hypolimnion (8 m) and sediments. Diversity is lowest in the photosynthetic plate (7 m). Shallower depths (5 m, 7 m) are rich in Actinobacteria, Alphaproteobacteria, and Gammaproteo- bacteria, while deeper depths (8 m, sediments) are rich in Crenarchaeota, Natronoanaerobium, and Verrucomi- crobia. The heterogeneous distribution of Deltaproteobacteria and Epsilonproteobacteria between 7 and 8 m is consistent with metabolisms involving sulfur intermediates in the chemocline, but complete sulfate reduction in the hypolimnion. -
Unit 1 Lesson 4: Coral Reefs As Indicators of Paleoclimate
CORAL REEFS Unit 1 Lesson 4: Coral Reefs as Indicators of Paleoclimate esson Objectives: Students will gain knowledge of how the marine environment can tell a story about years past through naturally recorded geographic and environmental phenomenon. Vocabulary: Paleoclimate, greenhouse effect, proxy data information gathered from www.noaa.gov Paleoclimatology is the study temperature increases may of the weather and climate from have a natural cause, for ages past. The word is derived example, from elevated from the Greek root word volcanic activity. "paleo-," which means "long ago" with combined with Gases in the earth’s "climate," meaning weather. atmosphere which trap heat, Scientists and meteorologists and cause an increase in have been using instruments to temperature cause the measure climate and weather greenhouse effect. Carbon for only the past 140 years! dioxide (CO2), water vapor, How do they determine what and other gases in the the Earth's climate was like atmosphere absorb the infrared before then? They use rays forming a kind of blanket historical evidence called proxy around the earth. Scientists data. Examples of proxy data fear that if humans continue to include tree rings, old farmer’s place too much carbon dioxide diaries, ice cores, frozen pollen in the atmosphere, too much and ocean sediments. heat will be trapped, causing the global temperature to rise Scientists know the Earth's and resulting in devastating average temperature has effects. increased approximately 1°F since 1860. Is this warming Some scientists speculate that due to something people are natural events like volcanic releasing into the atmosphere eruptions or an increase in the or natural causes? Many sun's output, may be people today are quick to influencing the climate.