Design, Synthesis, and Evaluation of Cysteine Protease Inhibitors
Total Page:16
File Type:pdf, Size:1020Kb
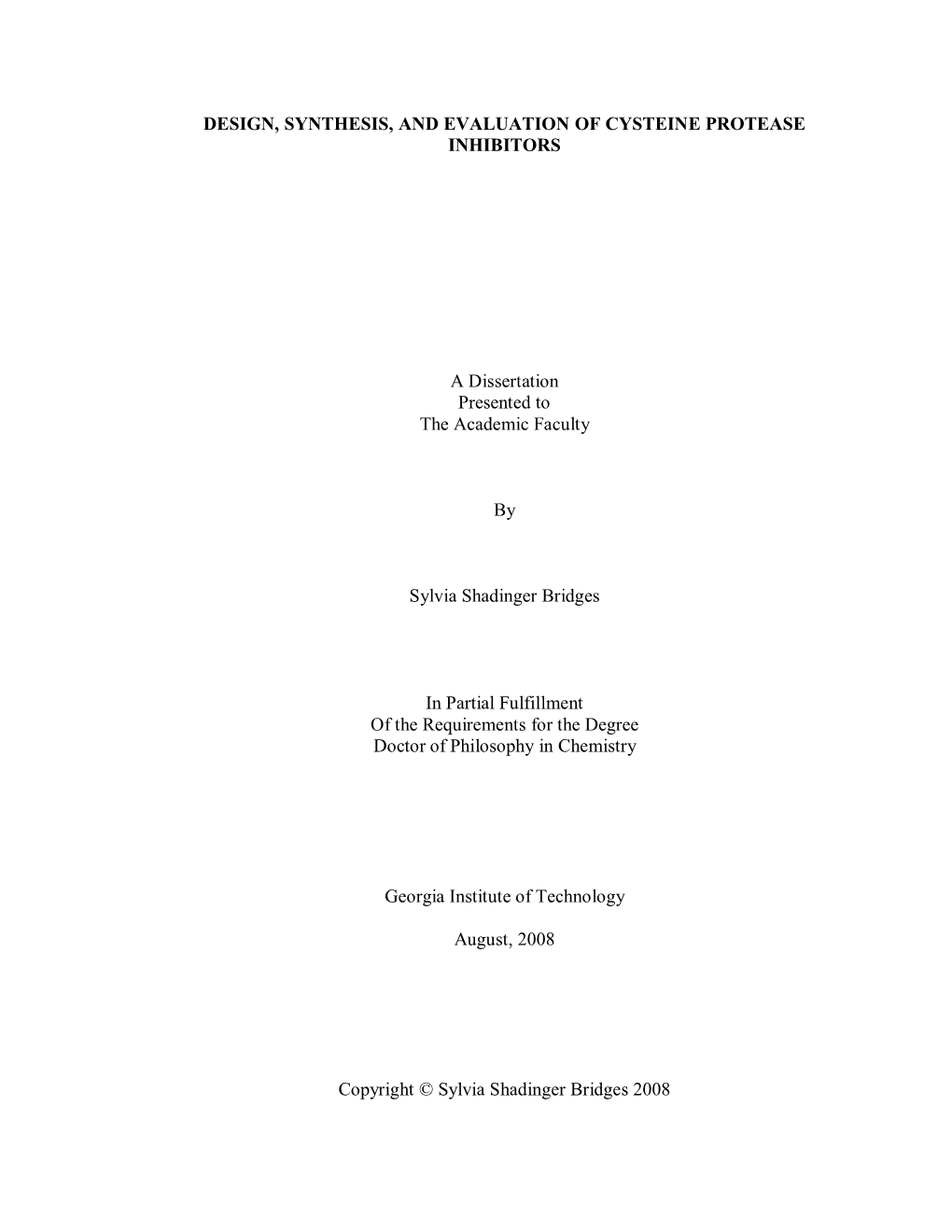
Load more
Recommended publications
-
Oleoresins and Naturally Occurring Compounds of Copaifera Genus As
www.nature.com/scientificreports OPEN Oleoresins and naturally occurring compounds of Copaifera genus as antibacterial and antivirulence agents against periodontal pathogens Fariza Abrão1, Thayná Souza Silva1, Claudia L. Moura1, Sérgio Ricardo Ambrósio2, Rodrigo Cassio Sola Veneziani2, Raphael E. F. de Paiva3, Jairo Kenupp Bastos4 & Carlos Henrique Gomes Martins1,5* Invasion of periodontal tissues by Porphyromonas gingivalis and Aggregatibacter actinomycetemcomitans can be associated with aggressive forms of periodontitis. Oleoresins from diferent copaifera species and their compounds display various pharmacological properties. The present study evaluates the antibacterial and antivirulence activity of oleoresins obtained from diferent copaifera species and of ten isolated compounds against two causative agents of periodontitis. The following assays were performed: determination of the minimum inhibitory concentration (MIC), determination of the minimum bactericidal concentration (MBC), and determination of the antibioflm activity by inhibition of bioflm formation and bioflm eradication tests. The antivirulence activity was assessed by hemagglutination, P. gingivalis Arg-X and Lis-X cysteine protease inhibition assay, and A. actinomycetemcomitans leukotoxin inhibition assay. The MIC and MBC of the oleoresins and isolated compounds 1, 2, and 3 ranged from 1.59 to 50 μg/ mL against P. gingivalis (ATCC 33277) and clinical isolates and from 6.25 to 400 μg/mL against A. actinomycetemcomitans (ATCC 43717) and clinical isolates. About the antibioflm activity, the oleoresins and isolated compounds 1, 2, and 3 inhibited bioflm formation by at least 50% and eradicated pre-formed P. gingivalis and A. actinomycetemcomitans bioflms in the monospecies and multispecies modes. A promising activity concerning cysteine protease and leucotoxin inhibition was also evident. In addition, molecular docking analysis was performed. -
Quercetin Inhibits Virulence Properties of Porphyromas Gingivalis In
www.nature.com/scientificreports OPEN Quercetin inhibits virulence properties of Porphyromas gingivalis in periodontal disease Zhiyan He1,2,3,7, Xu Zhang1,2,3,7, Zhongchen Song2,3,4, Lu Li5, Haishuang Chang6, Shiliang Li5* & Wei Zhou1,2,3* Porphyromonas gingivalis is a causative agent in the onset and progression of periodontal disease. This study aims to investigate the efects of quercetin, a natural plant product, on P. gingivalis virulence properties including gingipain, haemagglutinin and bioflm formation. Antimicrobial efects and morphological changes of quercetin on P. gingivalis were detected. The efects of quercetin on gingipains activities and hemolytic, hemagglutination activities were evaluated using chromogenic peptides and sheep erythrocytes. The bioflm biomass and metabolism with diferent concentrations of quercetin were assessed by the crystal violet and MTT assay. The structures and thickness of the bioflms were observed by confocal laser scanning microscopy. Bacterial cell surface properties including cell surface hydrophobicity and aggregation were also evaluated. The mRNA expression of virulence and iron/heme utilization was assessed using real time-PCR. Quercetin exhibited antimicrobial efects and damaged the cell structure. Quercetin can inhibit gingipains, hemolytic, hemagglutination activities and bioflm formation at sub-MIC concentrations. Molecular docking analysis further indicated that quercetin can interact with gingipains. The bioflm became sparser and thinner after quercetin treatment. Quercetin also modulate cell surface hydrophobicity and aggregation. Expression of the genes tested was down-regulated in the presence of quercetin. In conclusion, our study demonstrated that quercetin inhibited various virulence factors of P. gingivalis. Periodontal disease is a common chronic infammatory disease that characterized swelling and bleeding of the gums clinically, and leading to the progressive destruction of tooth-supporting tissues including the gingiva, alveolar bone, periodontal ligament, and cementum. -
Propeptide-Mediated Inhibition of Cognate Gingipain Proteinases
Propeptide-Mediated Inhibition of Cognate Gingipain Proteinases N. Laila Huq, Christine A. Seers, Elena C. Y. Toh, Stuart G. Dashper, Nada Slakeski, Lianyi Zhang, Brent R. Ward, Vincent Meuric, Dina Chen, Keith J. Cross, Eric C. Reynolds* Oral Health Cooperative Research Centre, Melbourne Dental School, Bio21 Institute of Molecular Science and Biotechnology, The University of Melbourne, Victoria, Australia Abstract Porphyromonas gingivalis is a major pathogen associated with chronic periodontitis. The organism’s cell-surface cysteine proteinases, the Arg-specific proteinases (RgpA, RgpB) and the Lys-specific proteinase (Kgp), which are known as gingipains have been implicated as major virulence factors. All three gingipain precursors contain a propeptide of around 200 amino acids in length that is removed during maturation. The aim of this study was to characterize the inhibitory potential of the Kgp and RgpB propeptides against the mature cognate enzymes. Mature Kgp was obtained from P. gingivalis mutant ECR368, which produces a recombinant Kgp with an ABM1 motif deleted from the catalytic domain (rKgp) that enables the otherwise membrane bound enzyme to dissociate from adhesins and be released. Mature RgpB was obtained from P. gingivalis HG66. Recombinant propeptides of Kgp and RgpB were produced in Escherichia coli and purified using nickel- affinity chromatography. The Kgp and RgpB propeptides displayed non-competitive inhibition kinetics with Ki values of 2.04 mM and 12 nM, respectively. Both propeptides exhibited selectivity towards their cognate proteinase. The specificity of both propeptides was demonstrated by their inability to inhibit caspase-3, a closely related cysteine protease, and papain that also has a relatively long propeptide. -
Role of Coagulation Factor 2 Receptor During Respiratory Pneumococcal Infections
Journal of Bacteriology and Virology 2016. Vol. 46, No. 4 p.319 – 325 http://dx.doi.org/10.4167/jbv.2016.46.4.319 Research Update (Minireview) Role of Coagulation Factor 2 Receptor during Respiratory Pneumococcal Infections * Seul Gi Shin1, Younghoon Bong2 and Jae Hyang Lim1 1Department of Microbiology, School of Medicine, Ewha Womans University, Seoul; 2College of Veterinary Medicine, Chonnam National University, Gwangju, Korea Coagulation factor 2 receptor (F2R), also well-known as a protease-activated receptor 1 (PAR1), is the first known thrombin receptor and plays a critical role in transmitting thrombin-mediated activation of intracellular signaling in many types of cells. It has been known that bacterial infections lead to activation of coagulation systems, and recent studies suggest that PAR1 may be critically involved not only in mediating bacteria-induced detrimental coagulation, but also in innate immune and inflammatory responses. Community-acquired pneumonia, which is frequently caused by Streptococcus pneumoniae (S. pneumoniae), is characterized as an intra-alveolar coagulation and an interstitial neutrophilic inflammation. Recently, the role of PAR1 in regulating pneumococcal infections has been proposed. However, the role of PAR1 in pneumococcal infections has not been clearly understood yet. In this review, recent findings on the role of PAR1 in pneumococcal infections and possible underlying molecular mechanisms by which S. pneumoniae regulates PAR1- mediated immune and inflammatory responses will be discussed. Key Words: Streptococcus pneumoniae, Coagulation factor 2 receptor, F2R, Protease-activated receptor 1, PAR1 mation (8). Lung injury at the early stage of infection is INTRODUCTION critical step to initiate intravascular dissemination of pneumo- coccus and develop detrimental systemic infections, such as Community-acquired pneumonia (CAP) is a major cause bacteremia, meningitis, arthritis, and septicemia (8). -
Serine Proteases with Altered Sensitivity to Activity-Modulating
(19) & (11) EP 2 045 321 A2 (12) EUROPEAN PATENT APPLICATION (43) Date of publication: (51) Int Cl.: 08.04.2009 Bulletin 2009/15 C12N 9/00 (2006.01) C12N 15/00 (2006.01) C12Q 1/37 (2006.01) (21) Application number: 09150549.5 (22) Date of filing: 26.05.2006 (84) Designated Contracting States: • Haupts, Ulrich AT BE BG CH CY CZ DE DK EE ES FI FR GB GR 51519 Odenthal (DE) HU IE IS IT LI LT LU LV MC NL PL PT RO SE SI • Coco, Wayne SK TR 50737 Köln (DE) •Tebbe, Jan (30) Priority: 27.05.2005 EP 05104543 50733 Köln (DE) • Votsmeier, Christian (62) Document number(s) of the earlier application(s) in 50259 Pulheim (DE) accordance with Art. 76 EPC: • Scheidig, Andreas 06763303.2 / 1 883 696 50823 Köln (DE) (71) Applicant: Direvo Biotech AG (74) Representative: von Kreisler Selting Werner 50829 Köln (DE) Patentanwälte P.O. Box 10 22 41 (72) Inventors: 50462 Köln (DE) • Koltermann, André 82057 Icking (DE) Remarks: • Kettling, Ulrich This application was filed on 14-01-2009 as a 81477 München (DE) divisional application to the application mentioned under INID code 62. (54) Serine proteases with altered sensitivity to activity-modulating substances (57) The present invention provides variants of ser- screening of the library in the presence of one or several ine proteases of the S1 class with altered sensitivity to activity-modulating substances, selection of variants with one or more activity-modulating substances. A method altered sensitivity to one or several activity-modulating for the generation of such proteases is disclosed, com- substances and isolation of those polynucleotide se- prising the provision of a protease library encoding poly- quences that encode for the selected variants. -
Science Journals
SCIENCE ADVANCES | RESEARCH ARTICLE HEALTH AND MEDICINE Copyright © 2019 The Authors, some rights reserved; Porphyromonas gingivalis in Alzheimer’s disease brains: exclusive licensee American Association Evidence for disease causation and treatment with for the Advancement of Science. No claim to small-molecule inhibitors original U.S. Government Stephen S. Dominy1*†, Casey Lynch1*, Florian Ermini1, Malgorzata Benedyk2,3, Agata Marczyk2, Works. Distributed 1 1 1 1 1 under a Creative Andrei Konradi , Mai Nguyen , Ursula Haditsch , Debasish Raha , Christina Griffin , Commons Attribution 1 1 1 1 4 Leslie J. Holsinger , Shirin Arastu-Kapur , Samer Kaba , Alexander Lee , Mark I. Ryder , License 4.0 (CC BY). Barbara Potempa5, Piotr Mydel2,6, Annelie Hellvard3,6, Karina Adamowicz2, Hatice Hasturk7,8, Glenn D. Walker9, Eric C. Reynolds9, Richard L. M. Faull10, Maurice A. Curtis11,12, Mike Dragunow11,13, Jan Potempa2,5* Porphyromonas gingivalis, the keystone pathogen in chronic periodontitis, was identified in the brain of Alzheimer’s disease patients. Toxic proteases from the bacterium called gingipains were also identified in the brain of Alzheimer’s Downloaded from patients, and levels correlated with tau and ubiquitin pathology. Oral P. gingivalis infection in mice resulted in brain colonization and increased production of Ab1–42, a component of amyloid plaques. Further, gingipains were neurotoxic in vivo and in vitro, exerting detrimental effects on tau, a protein needed for normal neuronal func- tion. To block this neurotoxicity, we designed and synthesized small-molecule inhibitors targeting gingipains. Gingipain inhibition reduced the bacterial load of an established P. gingivalis brain infection, blocked Ab1–42 pro- duction, reduced neuroinflammation, and rescued neurons in the hippocampus. -
Porphyromonas Gingivalis Laboratory Strains and Clinical Isolates Exhibit Different Distribution of Cell Surface and Secreted Gingipains Christine A
JOURNAL OF ORAL MICROBIOLOGY 2020, VOL. 13, 1858001 https://doi.org/10.1080/20002297.2020.1858001 Porphyromonas gingivalis laboratory strains and clinical isolates exhibit different distribution of cell surface and secreted gingipains Christine A. Seers, A. Sayeed M. Mahmud, N. Laila Huq, Keith J. Cross and Eric C. Reynolds Oral Health Cooperative Research Centre, Melbourne Dental School, Bio21 Institute, The University of Melbourne, Melbourne, Australia ABSTRACT ARTICLE HISTORY Background: The cell-surface cysteine proteinases RgpA, RgpB (Arg-gingipain), and Kgp (Lys- Received 16 July 2020 gingipain) are major virulence factors of P. gingivalis, a keystone pathogen in the develop Revised 26 November 2020 Accepted 27 November ment of destructive periodontal disease. The gingipains function as proteinases and trans 2020 peptidases utilising small peptides such as glycylglycine as acceptor molecules. However, the characteristics of the gingipains from most P. gingivalis strains have not been determined. KEYWORDS Methods: We determined the phenotypes of a panel of P. gingivalis laboratory strains and Porphyromonas gingivalis; global clinical isolates with respect to growth on blood agar plus whole-cell and vesicle-free gingipains; propeptides; culture supernatant (VFSN) Arg- and Lys-specific proteinase activities. protease; proteinase; Results: The P. gingivalis isolates exhibited different growth characteristics and hydrolysis of periodontitis haemoglobin in solid media. Whole-cell Arg-gingipain Vmax varied 5.8-fold and the whole cell Lys-gingipain Vmax varied 2.1-fold across the strains. Furthermore, the P. gingivalis strains showed more than 107-fold variance in soluble Arg-gingipain activity in VFSN and more than 371-fold variance in soluble Lys-gingipain activity in VFSN. -
The Role of Tannerella Forsythia and Porphyromonas Gingivalis in Pathogenesis of Esophageal Cancer Bartosz Malinowski1, Anna Węsierska1, Klaudia Zalewska1, Maya M
Malinowski et al. Infectious Agents and Cancer (2019) 14:3 https://doi.org/10.1186/s13027-019-0220-2 REVIEW Open Access The role of Tannerella forsythia and Porphyromonas gingivalis in pathogenesis of esophageal cancer Bartosz Malinowski1, Anna Węsierska1, Klaudia Zalewska1, Maya M. Sokołowska1, Wiktor Bursiewicz1*, Maciej Socha3, Mateusz Ozorowski1, Katarzyna Pawlak-Osińska2 and Michał Wiciński1 Abstract Tannerella forsythia and Porphyromonas gingivalis are anaerobic, Gram-negative bacterial species which have been implicated in periodontal diseases as a part of red complex of periodontal pathogens. Esophageal cancer is the eight most common cause of cancer deaths worldwide. Higher rates of esophageal cancer cases may be attributed to lifestyle factors such as: diet, obesity, alcohol and tobacco use. Moreover, the presence of oral P. gingivalis and T. forsythia has been found to be associated with an increased risk of esophageal cancer. Our review describes theroleofP. gingivalis and T. forsythia in signaling pathways responsible for cancer development. It has been shown that T. forsythia may induce pro-inflammatory cytokines such as IL-1β and IL-6 by CD4 + T helper cells and TNF-α. Moreover, gingipain K produced by P. gingivalis, affects hosts immune system by degradation of immunoglobulins and complement system (C3 and C5 components). Discussed bacteria are responsible for overexpression of MMP-2, MMP-2 and GLUT transporters. Keywords: Esophageal cancer, Tannerella forsythia, Porphyromonas gingivalis Background of cases) and adenocarcinoma (10%). Currently, there is a Cancer is a significant problem in the modern world. It downward trend in the incidence of squamous cell carcin- concerns the entire population. In developed countries, oma and an increase in adenocarcinoma [1]. -
Porphyromonas Gingivalis Is a Strong Risk Factor for Alzheimer's Disease
Journal of Alzheimer’s Disease Reports 4 (2020) 501–511 501 DOI 10.3233/ADR-200250 IOS Press Review Porphyromonas gingivalis is a Strong Risk Factor for Alzheimer’s Disease Shalini Kanagasingama, Sasanka S. Chukkapallib, Richard Welburya and Sim K. Singhraoa,∗ aBrain and Behavior Centre, Faculty of Clinical and Biomedical Sciences, School of Dentistry, University of Central Lancashire, Preston, UK bDepartment of Oral Biology, College of Dentistry, University of Florida, Gainesville, FL, USA Accepted 11 November 2020 Abstract. Porphyromonas gingivalis (P. gingivalis) is one of the several important bacterial pathogens associated with the sporadic Alzheimer’s disease (AD). Different serotypes are either capsulated or are non-capsulated. It has been demonstrated that P. gingivalis (non-capsulated) can reproduce the neurodegenerative AD-like changes in vitro, and a capsular P. gingivalis (strain W83) could reproduce the cardinal hallmark lesions of AD in a wild-type mouse model. All P. gingivalis forms express proteolytically active proteases that enable cleavage of the amyloid- protin precursor (APP) and tau resulting in the formation of amyloid- and neurofibrillary tangles. Tau is an established substrate for gingipains, which can cleave tau into various peptides. Some of the P. gingivalis fragmented tau protein peptides contain “VQIINK” and “VQIVYK” hexapeptide motifs which map to the flanking regions of the microtubule binding domains and are also found in paired helical filaments that form NFTs. P. gingivalis can induce peripheral inflammation in periodontitis and can also initiate signaling pathways that activate kinases, which in turn, phosphorylate neuronal tau. Periodontal disease related inflammation has metabolic implications for an individual’s peripheral and brain health as patients suffering from generalized periodontitis often have related co-morbidities and are “at risk” of developing AD. -
^ P X R, for the PURPOSES of INFORMATION ONLY
WORLD INTELLECTUAL PROPERTY ORGANIZATION PCT International Bureau INTERNATIONAL APPLICATION PUBLISHED UNDER THE PATENT COOPERATION TREATY (PCT) (51) International Patent Classification 6 : (11) International Publication Number: WO 98/49190 C07K 5/06, 5/08, 5/10 A l (43) International Publication Date: 5 November 1998 (05.11.98) (21) International Application Number: PCT/US98/08259 (74) Agents: BURKE, John, E. et al.; Cushman Darby & Cushman, Intellectual Property Group of Pillsbury Madison & Sutro, (22) International Filing Date: 24 April 1998 (24.04.98) 1100 New York Avenue, N.W., Washington, DC 20005 (US). (30) Priority Data: 60/044,819 25 April 1997 (25.04.97) US (81) Designated States: AL, AM, AT, AU, AZ, BA, BB, BG, BR, Not furnished 23 April 1998 (23.04.98) US BY, CA, CH, CN, CU, CZ, DE, DK, EE, ES, FI, GB, GE, GH, GM, GW, HU, ID, IL, IS, JP, KE, KG, KP, KR, KZ, LC, LK, LR, LS, LT, LU, LV, MD, MG, MK, MN, MW, (71) Applicant (for all designated States except US): CORTECH, MX, NO, NZ, PL, PT, RO, RU, SD, SE, SG, SI, SK, SL, INC. [US/US]; 6850 North Broadway, Denver, CO 80221 TJ, TM, TR, TT, UA, UG, US, UZ, VN, YU, ZW, ARIPO (US). patent (GH, GM, KE, LS, MW, SD, SZ, UG, ZW), Eurasian patent (AM, AZ, BY, KG, KZ, MD, RU, TJ, TM), European (72) Inventors; and patent (AT, BE, CH, CY, DE, DK, ES, FI, FR, GB, GR, (75) Inventors/Applicants(for US only): SPRUCE, Lyle, W. IE, IT, LU, MC, NL, PT, SE), OAPI patent (BF, BJ, CF, [US/US]; 948 Camino Del Sol, Chula Vista, CA 91910 CG, Cl, CM, GA, GN, ML, MR, NE, SN, TD, TG). -
Targeting a Cysteine Protease from a Pathobiont Alleviates Experimental
Peng et al. Arthritis Research & Therapy (2020) 22:114 https://doi.org/10.1186/s13075-020-02205-z RESEARCH ARTICLE Open Access Targeting a cysteine protease from a pathobiont alleviates experimental arthritis Hsin-Yi Peng1†, Shih-Yao Chen2†, Shih-Hong Siao3, Jinghua Tsai Chang4, Ting-Yin Xue1, Yi-Hsuan Lee1, Ming-Shiou Jan6, Gregory J. Tsay1,5*† and Moncef Zouali7,8,9*† Abstract Background: Several lines of evidence suggest that the pathobiont Porphyromonas gingivalis is involved in the development and/or progression of auto-inflammatory diseases. This bacterium produces cysteine proteases, such as gingipain RgpA, endowed with the potential to induce significant bone loss in model systems and in patients. Objective: We sought to gain further insight into the role of this pathobiont in rheumatoid arthritis (RA) and to identify novel therapeutic targets for auto-inflammatory diseases. Methods: We profiled the antibody response to RgPA-specific domains in patient sera. We also tested the potential protective effects of RgpA domains in an experimental arthritis model. Results: Pre-immunization of rats with purified recombinant RgpA domains alleviated arthritis in the joints of the rodents and reduced bone erosion. Using a functional genomics approach at both the mRNA and protein levels, we report that the pre-immunizations reduced arthritis severity by impacting a matrix metalloprotease characteristic of articular injury, a chemokine known to be involved in recruiting inflammatory cells, and three inflammatory cytokines. Finally, we identified an amino acid motif in the RgpA catalytic domain of P. gingivalis that shares sequence homology with type II collagen. Conclusion: We conclude that pre-immunization against gingipain domains can reduce the severity of experimentally induced arthritis. -
Caspases: Pharmacological Manipulation of Cell Death Inna N
Review series Caspases: pharmacological manipulation of cell death Inna N. Lavrik, Alexander Golks, and Peter H. Krammer Division of Immunogenetics, Tumor Immunology Program, German Cancer Research Center, Heidelberg, Germany. Caspases, a family of cysteine proteases, play a central role in apoptosis. During the last decade, major progress has been made to further understand caspase structure and function, providing a unique basis for drug design. This Review gives an overview of caspases and their classification, structure, and substrate specificity. We also describe the current knowledge of how interference with caspase signaling can be used to pharmacologically manipulate cell death. Introduction involved in the transduction of the apoptotic signal. This superfam- Apoptosis, or programmed cell death, is a common property of all ily consists of the death domain (DD), the death effector domain multicellular organisms (1, 2). It can be triggered by a number of (DED), and the caspase recruitment domain (CARD) (11). Each of factors, including ultraviolet or γ-irradiation, growth factor with- these motifs interacts with other proteins by homotypic interac- drawal, chemotherapeutic drugs, or signaling by death receptors tions. All members of the death domain superfamily are character- (DRs) (3, 4). The central role in the regulation and the execution of ized by similar structures that comprise 6 or 7 antiparallel amphipa- apoptotic cell death belongs to caspases (5–7). Caspases, a family thic α-helices. Structural similarity suggests a common evolutionary of cysteinyl aspartate–specific proteases, are synthesized as zymo- origin for all recruitment domains (12). However, the nature of the gens with a prodomain of variable length followed by a large sub- homotypic interactions differs within the superfamily.