Parallel Evolution Along an Ecological Gradient in African Cichlid
Total Page:16
File Type:pdf, Size:1020Kb
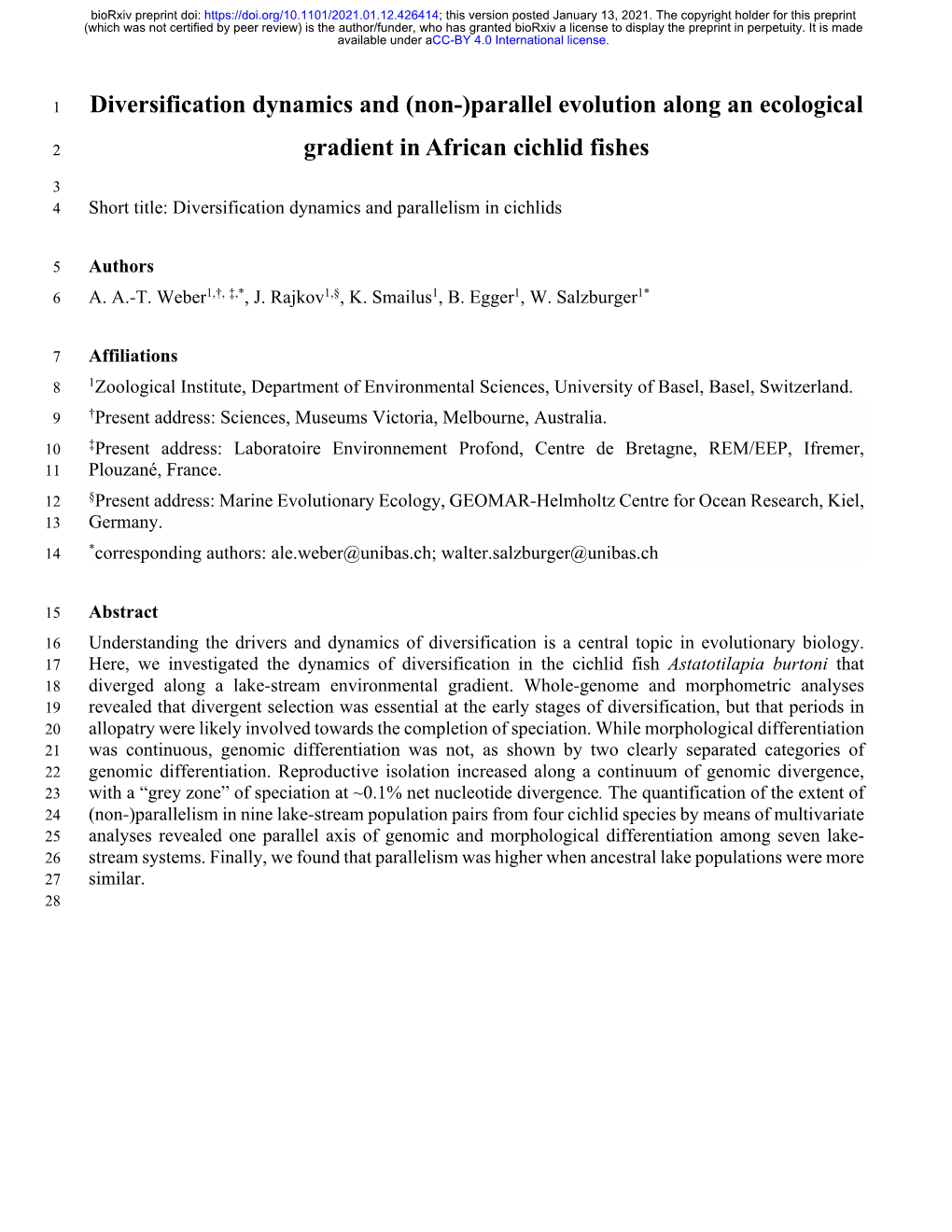
Load more
Recommended publications
-
View/Download
CICHLIFORMES: Cichlidae (part 3) · 1 The ETYFish Project © Christopher Scharpf and Kenneth J. Lazara COMMENTS: v. 6.0 - 30 April 2021 Order CICHLIFORMES (part 3 of 8) Family CICHLIDAE Cichlids (part 3 of 7) Subfamily Pseudocrenilabrinae African Cichlids (Haplochromis through Konia) Haplochromis Hilgendorf 1888 haplo-, simple, proposed as a subgenus of Chromis with unnotched teeth (i.e., flattened and obliquely truncated teeth of H. obliquidens); Chromis, a name dating to Aristotle, possibly derived from chroemo (to neigh), referring to a drum (Sciaenidae) and its ability to make noise, later expanded to embrace cichlids, damselfishes, dottybacks and wrasses (all perch-like fishes once thought to be related), then beginning to be used in the names of African cichlid genera following Chromis (now Oreochromis) mossambicus Peters 1852 Haplochromis acidens Greenwood 1967 acies, sharp edge or point; dens, teeth, referring to its sharp, needle-like teeth Haplochromis adolphifrederici (Boulenger 1914) in honor explorer Adolf Friederich (1873-1969), Duke of Mecklenburg, leader of the Deutsche Zentral-Afrika Expedition (1907-1908), during which type was collected Haplochromis aelocephalus Greenwood 1959 aiolos, shifting, changing, variable; cephalus, head, referring to wide range of variation in head shape Haplochromis aeneocolor Greenwood 1973 aeneus, brazen, referring to “brassy appearance” or coloration of adult males, a possible double entendre (per Erwin Schraml) referring to both “dull bronze” color exhibited by some specimens and to what -
Genome-Wide DNA Methylation and Long-Term Ambient Air Pollution
Lee et al. Clinical Epigenetics (2019) 11:37 https://doi.org/10.1186/s13148-019-0635-z RESEARCH Open Access Genome-wide DNA methylation and long- term ambient air pollution exposure in Korean adults Mi Kyeong Lee1 , Cheng-Jian Xu2,3,4, Megan U. Carnes5, Cody E. Nichols1, James M. Ward1, The BIOS consortium, Sung Ok Kwon6, Sun-Young Kim7*†, Woo Jin Kim6*† and Stephanie J. London1*† Abstract Background: Ambient air pollution is associated with numerous adverse health outcomes, but the underlying mechanisms are not well understood; epigenetic effects including altered DNA methylation could play a role. To evaluate associations of long-term air pollution exposure with DNA methylation in blood, we conducted an epigenome- wide association study in a Korean chronic obstructive pulmonary disease cohort (N = 100 including 60 cases) using Illumina’s Infinium HumanMethylation450K Beadchip. Annual average concentrations of particulate matter ≤ 10 μmin diameter (PM10) and nitrogen dioxide (NO2) were estimated at participants’ residential addresses using exposure prediction models. We used robust linear regression to identify differentially methylated probes (DMPs) and two different approaches, DMRcate and comb-p, to identify differentially methylated regions (DMRs). Results: After multiple testing correction (false discovery rate < 0.05), there were 12 DMPs and 27 DMRs associated with PM10 and 45 DMPs and 57 DMRs related to NO2. DMP cg06992688 (OTUB2) and several DMRs were associated with both exposures. Eleven DMPs in relation to NO2 confirmed previous findings in Europeans; the remainder were novel. Methylation levels of 39 DMPs were associated with expression levels of nearby genes in a separate dataset of 3075 individuals. -
Presentation
Evolution in Darwin’s Dreampond: The genomic substrate for adaptive radiation in Lake Tanganyika cichlid fish Walter Salzburger Zoological Institute drawings: Julie Johnson drawings: !Charles R. Darwin’s (1809-1882) journey onboard of the HMS Beagle lasted from 27 December 1831 until 2 October 1836 Adaptive Radiation !Darwin’s specimens were classified as “an entirely new group” of 12 species by ornithologist John Gould (1804-1881) African Great Lakes taxonomic diversity at the species level L. Turkana 1 5 50 500 species 0 50 100 % endemics 4°N L. Tanganyika L. Tanganyika L. Albert 2°N L. Malawi L. Malawi L. Edward L. Victoria L. Victoria 0° L. Edward L. Edward L. Kivu L. Victoria 2°S L. Turkana L. Turkana L. Albert L. Albert 4°S L. Kivu L. Kivu L. Tanganyika 6°S taxonomic diversity at the genus level 10 20 30 40 50 genera 0 50 100 % endemics 8°S Rungwe L. Tanganyika L. Tanganyika Volcanic Field L. Malawi 10°S L. Malawi L. Victoria L. Victoria 12°S L. Malawi L. Edward L. Edward L. Turkana L. Turkana 14°S cichlid fish non-cichlid fish L. Albert L. Albert gastropods bivalves 28°E 30°E 32°E 34°E 36°E L. Kivu L. Kivu ostracods ••• W Salzburger, B Van Bockxlaer, AS Cohen (2017), AREES | AS Cohen & W Salzburger (2017) Scientific Drilling Cichlid Fishes Fotos: Angel M. Fitor Angel M. Fotos: !About every 20th fish species on our planet is the product of the ongoing explosive radiations of cichlids in the East African Great Lakes taxonomic~Diversity Victoria [~500 sp.] Tanganyika [250 sp.] Malawi [~1000 sp.] ••• ME Santos & W Salzburger (2012) Science ecological morphological~Diversity zooplanktivore insectivore piscivore algae scraper leaf eater fin biter eye biter mud digger scale eater ••• H Hofer & W Salzburger (2008) Biologie III ecological morphological~Diversity ••• W Salzburger (2009) Molecular Ecology astbur Astbur.:1-90001 Alignment 1 neobri 100% Neobri. -
A Chromosome Level Genome of Astyanax Mexicanus Surface Fish for Comparing Population
bioRxiv preprint doi: https://doi.org/10.1101/2020.07.06.189654; this version posted July 6, 2020. The copyright holder for this preprint (which was not certified by peer review) is the author/funder. All rights reserved. No reuse allowed without permission. 1 Title 2 A chromosome level genome of Astyanax mexicanus surface fish for comparing population- 3 specific genetic differences contributing to trait evolution. 4 5 Authors 6 Wesley C. Warren1, Tyler E. Boggs2, Richard Borowsky3, Brian M. Carlson4, Estephany 7 Ferrufino5, Joshua B. Gross2, LaDeana Hillier6, Zhilian Hu7, Alex C. Keene8, Alexander Kenzior9, 8 Johanna E. Kowalko5, Chad Tomlinson10, Milinn Kremitzki10, Madeleine E. Lemieux11, Tina 9 Graves-Lindsay10, Suzanne E. McGaugh12, Jeff T. Miller12, Mathilda Mommersteeg7, Rachel L. 10 Moran12, Robert Peuß9, Edward Rice1, Misty R. Riddle13, Itzel Sifuentes-Romero5, Bethany A. 11 Stanhope5,8, Clifford J. Tabin13, Sunishka Thakur5, Yamamoto Yoshiyuki14, Nicolas Rohner9,15 12 13 Authors for correspondence: Wesley C. Warren ([email protected]), Nicolas Rohner 14 ([email protected]) 15 16 Affiliation 17 1Department of Animal Sciences, Department of Surgery, Institute for Data Science and 18 Informatics, University of Missouri, Bond Life Sciences Center, Columbia, MO 19 2 Department of Biological Sciences, University of Cincinnati, Cincinnati, OH 20 3 Department of Biology, New York University, New York, NY 21 4 Department of Biology, The College of Wooster, Wooster, OH 22 5 Harriet L. Wilkes Honors College, Florida Atlantic University, Jupiter FL 23 6 Department of Genome Sciences, University of Washington, Seattle, WA 1 bioRxiv preprint doi: https://doi.org/10.1101/2020.07.06.189654; this version posted July 6, 2020. -
Hered 347 Master..Hered 347 .. Page702
Heredity 80 (1998) 702–714 Received 3 June 1997 Phylogeny of African cichlid fishes as revealed by molecular markers WERNER E. MAYER*, HERBERT TICHY & JAN KLEIN Max-Planck-Institut f¨ur Biologie, Abteilung Immungenetik, Corrensstr. 42, D-72076 T¨ubingen, Germany The species flocks of cichlid fish in the three great East African Lakes, Victoria, Malawi, and Tanganyika, have arisen in each lake by explosive adaptive radiation. Various questions concerning their phylogeny have not yet been answered. In particular, the identity of the ancestral founder species and the monophyletic origin of the haplochromine cichlids from the East African lakes have not been established conclusively. In the present study, we used the anonymous nuclear DNA marker DXTU1 as a step towards answering these questions. A 280 bp-fragment of the DXTU1 locus was amplified by the polymerase chain reaction from East African lacustrine species, the East African riverine cichlid species Haplochromis bloyeti, H. burtoni and H. sparsidens, and other African cichlids. Sequencing revealed several indels and substitutions that were used as cladistically informative markers to support a phylogenetic tree constructed by the neighbor-joining method. The topology, although not supported by high bootstrap values, corresponds well to the geographical distribution and previous classifica- tion of the cichlids. Markers could be defined that: (i) differentiate East African from West African cichlids; (ii) distinguish the riverine and Lake Victoria/Malawi haplochromines from Lake Tanganyika cichlids; and (iii) indicate the existence of a monophyletic Lake Victoria cichlid superflock which includes haplochromines from satellite lakes and East African rivers. In order to resolve further the relationship of East African riverine and lacustrine species, mtDNA cytochrome b and control region segments were sequenced. -
28. Annexe 2 Haplochromis
Description de ‘Haplochromis’ snoeksi ‘Haplochromis’ snoeksi (Perciformes: Cichlidae) a new species from the Inkisi River basin, Lower Congo Soleil WAMUINI L. *, ** Emmanuel VREVEN *** and Pierre VANDEWALLE** * I.S.P. Mbanza-Ngungu, Département de Biologie, B.P. 127 - Mbanza-Ngungu, Democratic Republic of Congo (DRC). E-mail: [email protected] ** Université de Liège, Faculté des Sciences, Laboratoire de Morphologie fonctionnelle et évolutive, B-4000 Liège, Belgium. E-mail: [email protected] *** Royal Museum for Central Africa, Vertebrate Section, Ichthyology, B-3080 Tervuren, Belgium; E-mail: [email protected] Abstract ‘Haplochromis’ snoeksi , new species, is described from a single locality, Ngeba village on the Ngeba /Ngufu River, a right bank affluent of the Inkisi River basin (Lower Congo; Democratic Republic of Congo). The species is distinguished from its riverine Congo River basin congeners by the following unique combination of characters: thorax partially naked, i.e. without small scales; 16 scales around caudal peduncle; and an upper lateral line with 23 scales. Résumé Une nouvelle espèce, ‘ Haplochromis ’ snoeksi , est décrite d’une seule localité, le village Ngeba sur la rivière Ngeba/Ngufu, un affluent de la rive droite de l’Inkisi (Bas-Congo; République Démocratique du Congo). Cette espèce se distingue des ses congénères des rivières de la province ichtyologiques du bassin du Congo par la combinaison des caractères suivants : un espace thoracique partiellement nu, c'est-à-dire dépourvu d’écailles, 16 écailles autour du pédoncule caudal et une ligne latéral supérieure avec 23 écailles. Introduction The family Cichlidae is characterized by a single nostril on either side of the head, a lateral line which is divided in two parts and a single continuous dorsal fin which has a spiny anterior and soft posterior fin-ray part (Snoeks, 1994; Nelson, 2006; Stiassny et al ., 2008). -
Out of Lake Tanganyika: Endemic Lake Fishes Inhabit Rapids of the Lukuga River
355 Ichthyol. Explor. Freshwaters, Vol. 22, No. 4, pp. 355-376, 5 figs., 3 tabs., December 2011 © 2011 by Verlag Dr. Friedrich Pfeil, München, Germany – ISSN 0936-9902 Out of Lake Tanganyika: endemic lake fishes inhabit rapids of the Lukuga River Sven O. Kullander* and Tyson R. Roberts** The Lukuga River is a large permanent river intermittently serving as the only effluent of Lake Tanganyika. For at least the first one hundred km its water is almost pure lake water. Seventy-seven species of fish were collected from six localities along the Lukuga River. Species of cichlids, cyprinids, and clupeids otherwise known only from Lake Tanganyika were identified from rapids in the Lukuga River at Niemba, 100 km from the lake, whereas downstream localities represent a Congo River fish fauna. Cichlid species from Niemba include special- ized algal browsers that also occur in the lake (Simochromis babaulti, S. diagramma) and one invertebrate picker representing a new species of a genus (Tanganicodus) otherwise only known from the lake. Other fish species from Niemba include an abundant species of clupeid, Stolothrissa tanganicae, otherwise only known from Lake Tangan- yika that has a pelagic mode of life in the lake. These species demonstrate that their adaptations are not neces- sarily dependent upon the lake habitat. Other endemic taxa occurring at Niemba are known to frequent vegetat- ed shore habitats or river mouths similar to the conditions at the entrance of the Lukuga, viz. Chelaethiops minutus (Cyprinidae), Lates mariae (Latidae), Mastacembelus cunningtoni (Mastacembelidae), Astatotilapia burtoni, Ctenochromis horei, Telmatochromis dhonti, and Tylochromis polylepis (Cichlidae). The Lukuga frequently did not serve as an ef- fluent due to weed masses and sand bars building up at the exit, and low water levels of Lake Tanganyika. -
A BAC Library of the East African Haplochromine Cichlid Fish
JOURNAL OF EXPERIMENTAL ZOOLOGY (MOL DEV EVOL) 306B:35–44 (2006) A BAC Library of the East African Haplochromine Cichlid Fish Astatotilapia burtoni MICHAEL LANG1y, TSUTOMU MIYAKE2, INGO BRAASCH1, DEBORAH TINNEMORE2, NICOL SIEGEL1, WALTER SALZBURGER1, 2Ã 1Ã CHRIS T. AMEMIYA , AND AXEL MEYER 1Lehrstuhl fu¨r Zoologie und Evolutionsbiologie, Department of Biology, University of Konstanz, 78457 Konstanz, Germany 2Benaroya Research Institute at Virginia Mason, Seattle, Washington 98101 ABSTRACT A BAC library was constructed from Astatotilapia burtoni, a haplochromine cichlid that is found in Lake Tanganyika, East Africa, and its surrounding rivers. The library was generated from genomic DNA of blood cells and comprises 96,768 individual clones. Its median insert size is 150 kb and the coverage is expected to represent about 14 genome equivalents. The coverage evaluation was based on genome size estimates that were obtained by flow cytometry. In addition, hybridization screens with five probes largely corroborate the above coverage estimate, although the number of clones ranged from 5 to 22 authenticated clones per single copy probe. The BAC library described here is expected to be useful to the scientific community interested in cichlid genomics as an important resource to gain new insights into the rapid evolution of the great species diversity of haplochromine cichlid fishes. J. Exp. Zool. (Mol. Dev. Evol.) 306B:35– 44, 2006. r 2005 Wiley-Liss, Inc. The species flocks of cichlid fishes of the East rivers, swamp and marsh areas (Meyer et al., ’91; African Great Lakes Victoria, Tanganyika and Salzburger et al., 2002a, 2005; Verheyen et al., Malawi are extraordinary examples for explosive 2003). -
Cytogenetics of Gymnogeophagus Setequedas (Cichlidae: Geophaginae), with Comments on Its Geographical Distribution
Neotropical Ichthyology, 15(2): e160035, 2017 Journal homepage: www.scielo.br/ni DOI: 10.1590/1982-0224-20160035 Published online: 26 June 2017 (ISSN 1982-0224) Copyright © 2017 Sociedade Brasileira de Ictiologia Printed: 30 June 2017 (ISSN 1679-6225) Cytogenetics of Gymnogeophagus setequedas (Cichlidae: Geophaginae), with comments on its geographical distribution Leonardo M. Paiz1, Lucas Baumgärtner2, Weferson J. da Graça1,3, Vladimir P. Margarido1,2 and Carla S. Pavanelli1,3 We provide cytogenetic data for the threatened species Gymnogeophagus setequedas, and the first record of that species collected in the Iguaçu River, within the Iguaçu National Park’s area of environmental preservation, which is an unexpected occurrence for that species. We verified a diploid number of 2n = 48 chromosomes (4sm + 24st + 20a) and the presence of heterochromatin in centromeric and pericentromeric regions, which are conserved characters in the Geophagini. The multiple nucleolar organizer regions observed in G. setequedas are considered to be apomorphic characters in the Geophagini, whereas the simple 5S rDNA cistrons located interstitially on the long arm of subtelocentric chromosomes represent a plesiomorphic character. Because G. setequedas is a threatened species that occurs in lotic waters, we recommend the maintenance of undammed environments within its known area of distribution. Keywords: Chromosomes, Conservation, Iguaçu River, Karyotype, Paraná River. Fornecemos dados citogenéticos para a espécie ameaçada Gymnogeophagus setequedas, e o primeiro registro da espécie coletado no rio Iguaçu, na área de preservação ambiental do Parque Nacional do Iguaçu, a qual é uma área de ocorrência inesperada para esta espécie. Verificamos em G. setequedas 2n = 48 cromossomos (4sm + 24st + 20a) e heterocromatina presente nas regiões centroméricas e pericentroméricas, as quais indicam caracteres conservados em Geophagini. -
Astatotilapia Burtoni Sex Differences in a Novel Object Recognition
bioRxiv preprint doi: https://doi.org/10.1101/2020.08.03.234658; this version posted October 14, 2020. The copyright holder for this preprint (which was not certified by peer review) is the author/funder, who has granted bioRxiv a license to display the preprint in perpetuity. It is made available under aCC-BY-NC-ND 4.0 International license. 1 Equal performance but distinct behaviors: Astatotilapia burtoni sex differences in 2 a novel object recognition task and spatial maze 3 4 Kelly J. Wallace1 & Hans A. Hofmann1 5 1Department of Integrative Biology, University of Texas, Austin, TX, 78712, USA 6 Article Type: Research Article 7 8 Kelly J. Wallace ORCID: 0000-0002-2361-1213 9 Hans A. Hofmann ORCID: 0000-0002-3335-330X 10 11 Author for correspondence: Kelly J. Wallace 12 [email protected] 13 University of Texas at Austin, Department of Integrative Biology 14 1 University Station C0990 15 Austin, Texas 78712 16 1 bioRxiv preprint doi: https://doi.org/10.1101/2020.08.03.234658; this version posted October 14, 2020. The copyright holder for this preprint (which was not certified by peer review) is the author/funder, who has granted bioRxiv a license to display the preprint in perpetuity. It is made available under aCC-BY-NC-ND 4.0 International license. 17 Highlights 18 - To ask if sexual dimorphism in cognition is reduced in a species where both 19 sexes navigate highly fluctuating social environments, we assessed performance 20 and behavior of the cichlid fish Astatotilapia burtoni in a novel object recognition 21 task and a spatial maze. -
Limnological Study of Lake Tanganyika, Africa with Special Emphasis on Piscicultural Potentiality Lambert Niyoyitungiye
Limnological Study of Lake Tanganyika, Africa with Special Emphasis on Piscicultural Potentiality Lambert Niyoyitungiye To cite this version: Lambert Niyoyitungiye. Limnological Study of Lake Tanganyika, Africa with Special Emphasis on Piscicultural Potentiality. Biodiversity and Ecology. Assam University Silchar (Inde), 2019. English. tel-02536191 HAL Id: tel-02536191 https://hal.archives-ouvertes.fr/tel-02536191 Submitted on 9 Apr 2020 HAL is a multi-disciplinary open access L’archive ouverte pluridisciplinaire HAL, est archive for the deposit and dissemination of sci- destinée au dépôt et à la diffusion de documents entific research documents, whether they are pub- scientifiques de niveau recherche, publiés ou non, lished or not. The documents may come from émanant des établissements d’enseignement et de teaching and research institutions in France or recherche français ou étrangers, des laboratoires abroad, or from public or private research centers. publics ou privés. “LIMNOLOGICAL STUDY OF LAKE TANGANYIKA, AFRICA WITH SPECIAL EMPHASIS ON PISCICULTURAL POTENTIALITY” A THESIS SUBMITTED TO ASSAM UNIVERSITY FOR PARTIAL FULFILLMENT OF THE REQUIREMENT FOR THE DEGREE OF DOCTOR OF PHILOSOPHY IN LIFE SCIENCE AND BIOINFORMATICS By Lambert Niyoyitungiye (Ph.D. Registration No.Ph.D/3038/2016) Department of Life Science and Bioinformatics School of Life Sciences Assam University Silchar - 788011 India Under the Supervision of Dr.Anirudha Giri from Assam University, Silchar & Co-Supervision of Prof. Bhanu Prakash Mishra from Mizoram University, Aizawl Defence date: 17 September, 2019 To Almighty and merciful God & To My beloved parents with love i MEMBERS OF EXAMINATION BOARD iv Contents Niyoyitungiye, 2019 CONTENTS Page Numbers CHAPTER-I INTRODUCTION .............................................................. 1-7 I.1 Background and Motivation of the Study .......................................... -
View/Download
CICHLIFORMES: Cichlidae (part 2) · 1 The ETYFish Project © Christopher Scharpf and Kenneth J. Lazara COMMENTS: v. 4.0 - 30 April 2021 Order CICHLIFORMES (part 2 of 8) Family CICHLIDAE Cichlids (part 2 of 7) Subfamily Pseudocrenilabrinae African Cichlids (Abactochromis through Greenwoodochromis) Abactochromis Oliver & Arnegard 2010 abactus, driven away, banished or expelled, referring to both the solitary, wandering and apparently non-territorial habits of living individuals, and to the authors’ removal of its one species from Melanochromis, the genus in which it was originally described, where it mistakenly remained for 75 years; chromis, a name dating to Aristotle, possibly derived from chroemo (to neigh), referring to a drum (Sciaenidae) and its ability to make noise, later expanded to embrace cichlids, damselfishes, dottybacks and wrasses (all perch-like fishes once thought to be related), often used in the names of African cichlid genera following Chromis (now Oreochromis) mossambicus Peters 1852 Abactochromis labrosus (Trewavas 1935) thick-lipped, referring to lips produced into pointed lobes Allochromis Greenwood 1980 allos, different or strange, referring to unusual tooth shape and dental pattern, and to its lepidophagous habits; chromis, a name dating to Aristotle, possibly derived from chroemo (to neigh), referring to a drum (Sciaenidae) and its ability to make noise, later expanded to embrace cichlids, damselfishes, dottybacks and wrasses (all perch-like fishes once thought to be related), often used in the names of African cichlid genera following Chromis (now Oreochromis) mossambicus Peters 1852 Allochromis welcommei (Greenwood 1966) in honor of Robin Welcomme, fisheries biologist, East African Freshwater Fisheries Research Organization (Jinja, Uganda), who collected type and supplied ecological and other data Alticorpus Stauffer & McKaye 1988 altus, deep; corpus, body, referring to relatively deep body of all species Alticorpus geoffreyi Snoeks & Walapa 2004 in honor of British carcinologist, ecologist and ichthyologist Geoffrey Fryer (b.