A Palaeoecological Approach to Savanna Dynamics and Shrub Encroachment in Namibia ______
Total Page:16
File Type:pdf, Size:1020Kb
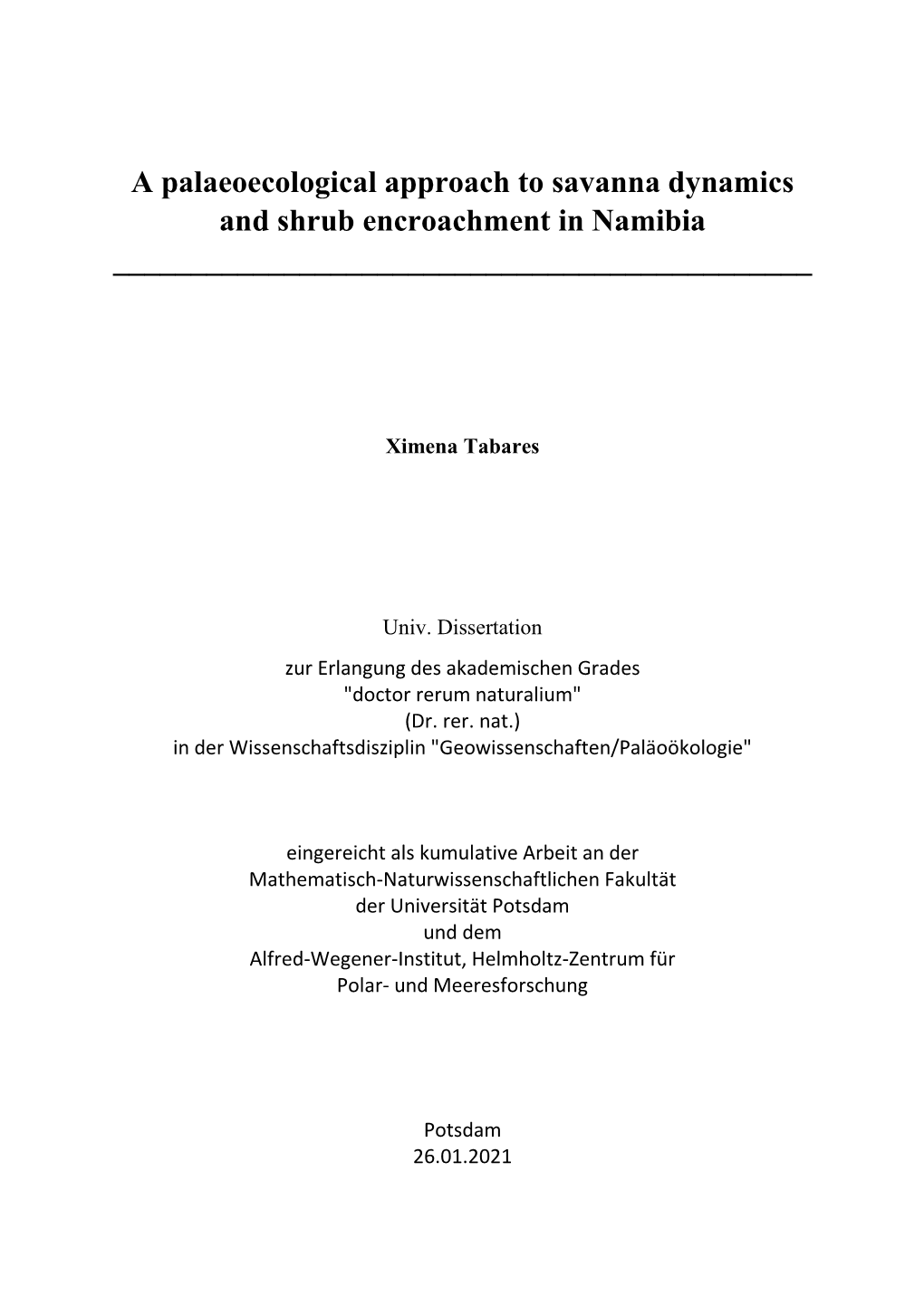
Load more
Recommended publications
-
Plants Used for Bone Fracture by Indigenous Folklore of Nizamabad District, Andhra Pradesh
International Multidisciplinary Research Journal 2012, 2(12):14-16 ISSN: 2231-6302 Available Online: http://irjs.info/ Plants used for bone fracture by Indigenous folklore of Nizamabad district, Andhra Pradesh Vijigiri Dinesh and P. P. Sharma* Department of Botany, Telangana University, Dichpally, Nizamabad -503322, India *Department of Botany, Muktanand College, Gangapur, Aurangabad – 431009 (Maharashtra), India Abstract The present investigation provides information on the therapeutic properties of 17 crude drugs used for treating bone fracture by the natives of Nizamabad District. Of these, 12 species are not reported earlier for the bone fracture in major literature published so far. Information on botanical name, vernacular name, family, part used, mode of medicine preparation and administration is provided. Keywords: Indigenous folklore, Nizamabad, Andhra Pradesh. INTRODUCTION observations and interviews with traditional healers (Viz. medicine Nizamabad district is situated in the northern part of the men, hakims and old aged people) and methodology used is based Andhra Pradesh and is one of the 10 districts of Telangana region in on the methods available in literature (Jain, 1989) and (Jain and the state of Andhra Pradesh. It lies between 18-5' and 19' of the Mudgal, 1999). northern latitudes, 77-40' and 78-37' of the eastern longitudes. The Ethnobotanical information about bone fracture gathered was geographical area is 7956 Sq. km’s i.e. 19,80,586 acres spread over documented in datasheets prepared. For collection of plant material, 923 villages in 36 mandals. Major rivers, such as, Godavari and local informer accompanied to authors. Plant identification was done Manjeera crosses Nizamabad district with some other streams by using regional flora and flora of adjoining districts (Pullaih and Kalyani, Kaulas, Peddavagu also exist in the district. -
Seasonal Selection Preferences for Woody Plants by Breeding Herds of African Elephants (Loxodonta Africana)In a Woodland Savanna
Hindawi Publishing Corporation International Journal of Ecology Volume 2013, Article ID 769587, 10 pages http://dx.doi.org/10.1155/2013/769587 Research Article Seasonal Selection Preferences for Woody Plants by Breeding Herds of African Elephants (Loxodonta africana)in a Woodland Savanna J. J. Viljoen,1 H. C. Reynecke,1 M. D. Panagos,1 W. R. Langbauer Jr.,2 and A. Ganswindt3,4 1 Department of Nature Conservation, Tshwane University of Technology, Private Bag X680, Pretoria 0001, South Africa 2 ButtonwoodParkZoo,NewBedford,MA02740,USA 3 Department of Zoology and Entomology, University of Pretoria, Pretoria 0002, South Africa 4 Department of Production Animal Studies, Faculty of Veterinary Science, University of Pretoria, Onderstepoort 0110, South Africa Correspondence should be addressed to J. J. Viljoen; [email protected] Received 19 November 2012; Revised 25 February 2013; Accepted 25 February 2013 Academic Editor: Bruce Leopold Copyright © 2013 J. J. Viljoen et al. This is an open access article distributed under the Creative Commons Attribution License, which permits unrestricted use, distribution, and reproduction in any medium, provided the original work is properly cited. To evaluate dynamics of elephant herbivory, we assessed seasonal preferences for woody plants by African elephant breeding herds in the southeastern part of Kruger National Park (KNP) between 2002 and 2005. Breeding herds had access to a variety of woody plants, and, of the 98 woody plant species that were recorded in the elephant’s feeding areas, 63 species were utilized by observed animals. Data were recorded at 948 circular feeding sites (radius 5 m) during wet and dry seasons. Seasonal preference was measured by comparing selection of woody species in proportion to their estimated availability and then ranked according to the Manly alpha () index of preference. -
Grewia Tenax
Academic Sciences Asian Journal of Pharmaceutical and Clinical Research Vol 5, Suppl 3, 2012 ISSN - 0974-2441 Review Article Vol. 4, Issue 3, 2011 Grewia tenax (Frosk.) Fiori.- A TRADITIONAL MEDICINAL PLANT WITH ENORMOUS ISSNECONOMIC - 0974-2441 PROSPECTIVES NIDHI SHARMA* AND VIDYA PATNI Department of Botany, University of Rajasthan, Jaipur- 302004, Rajasthan, India.Email: [email protected] Received:11 May 2012, Revised and Accepted:25 June 2012 ABSTRACT The plant Grewia tenax (Frosk.) Fiori. belonging to the family Tiliaceae, is an example of multipurpose plant species which is the source of food, fodder, fiber, fuelwood, timber and a range of traditional medicines that cure various perilous diseases and have mild antibiotic properties. The plant preparations are used for the treatment of bone fracture and for bone strengthening and tissue healing. The fruits are used for promoting fertility in females and are considered in special diets for pregnant women and anemic children. The plant is adapted to high temperatures and dry conditions and has deep roots which stabilize sand dunes. The shrubs play effectively for rehabilitation of wastelands. The plant parts are rich in amino acids and mineral elements and contain some pharmacologically active constituents. The plant is identified in trade for its fruits. Plant is also sold as wild species of medicinal and aromatic plant and is direct or indirect source of income for the tribal people. But the prolonged seed dormancy is a typical feature and vegetative propagation is not well characterized for the plant. Micropropagation by tissue culture techniques may play an effective role for plant conservation. -
Patterns of Plant Diversity and Endemism in Namibia
View metadata, citation and similar papers at core.ac.uk brought to you by CORE provided by Stellenbosch University SUNScholar Repository Bothalia 36,2: 175-189(2006) Patterns of plant diversity and endemism in Namibia P. CRAVEN* and P VORSTER** Keywords: Namibia, phytogeography, plant endemism ABSTRACT Species richness, endemism and areas that are rich in both species and endemic species were assessed and mapped for Namibia. High species diversity corresponds with zones where species overlap. These are particularly obvious where there are altitudinal variations and in high-lying areas. The endemic flora o f Namibia is rich and diverse. An estimated 16% of the total plant species in Namibia are endemic to the country. Endemics are in a wide variety o f families and sixteen genera are endemic. Factors that increase the likelihood o f endemism are mountains, hot deserts, diversity o f substrates and microclimates. The distribution of plants endemic to Namibia was arranged in three different ways. Firstly, based on a grid count with the phytogeographic value of the species being equal, overall endemism was mapped. Secondly, range restricted plant species were mapped individually and those with congruent distribution patterns were combined. Thirdly, localities that are important for very range-restricted species were identified. The resulting maps of endemism and diversity were compared and found to correspond in many localities. When overall endemism is compared with overall diversity, rich localities may consist o f endemic species with wide ranges. The other methods identify important localities with their own distinctive complement of species. INTRODUCTION (1994). It was based on distributional data per magiste rial district following Merxmiiller (1966-1972), as well Species diversity was traditionally measured by count as other literature. -
Dodonaea Triquetra
Plant of the Month - May by Allan Carr Dodonaea triquetra Forest Hop Bush Pronunciation: doh-doh-NEE-a try-QUET-ra SAPINDACEAE Derivation: Dononaea, after Rembert Dodoens, sixteenth century Flemish botanist whose name was latinized to Dodonaeus; triquetra, from the Latin, triquetris – triangular, a reference to the three-winged capsule. Leaves, fruits Male Flowers Female Flowers The genus Dodonaea has about 69 species of which 60 are endemic in Australia. This species was grown in England in 1790. As a group Dodonaeas have the common name hop bushes, gained from the decorative capsules resembling hops used in brewing beer. Description: D. triquetra is a small to medium open shrub to 3 m by 1.5 m found in the forest under storey from Bundaberg, Qld to Orbost, Vic. Young branchlets are reddish- brown and angular or flattened. Host plant for Fiery Jewel butterfly (Hypochrysops ignita). Leaves, to 120 mm by 35 mm are alternate, glossy dark green above and slightly paler below. They have a prominent central nerve and margins are entire to wavy. Some descriptions of shape from various regions are - ‘oblong to *lanceolate’, ‘*elliptic, sometimes *lanceolate or *ovate’, ‘tapering shortly to a point’ so it seems they can be quite variable. These leaves were chewed by Aboriginal people as a painkiller for toothache and chewed leaves were used in the treatment of stonefish and stringray wounds. Flowers are small and green in terminal *panicles on separate male and female plants. These inconspicuous flowers have no nectar to attract pollinators but the absence of petals allows pollen to be distributed by wind. -
Download Download
Botswana Journal of Agriculture and Applied Sciences, Volume 14, Issue 1 (2020) 7–16 BOJAAS Research Article Comparative nutritive value of an invasive exotic plant species, Prosopis glandulosa Torr. var. glandulosa, and five indigenous plant species commonly browsed by small stock in the BORAVAST area, south-western Botswana M. K. Ditlhogo1, M. P Setshogo1,* and G. Mosweunyane2 1Department of Biological Sciences, University of Botswana, Private Bag UB00704, Gaborone, Botswana. 2Geoflux Consulting Company, P.O. Box 2403, Gaborone, Botswana. ARTICLE INFORMATION ________________________ Keywords Abstract: Nutritive value of an invasive exotic plant species, Prosopis glandulosa Torr. var. glandulosa, and five indigenous plant species Nutritive value commonly browsed by livestock in Bokspits, Rapplespan, Vaalhoek and Prosopis glandulosa Struizendam (BORAVAST), southwest Botswana, was determined and BORAVAST compared. These five indigenous plant species were Vachellia Indigenous plant species hebeclada (DC.) Kyal. & Boatwr. subsp. hebeclada, Vachellia erioloba (E. Mey.) P.J.H. Hurter, Senegalia mellifera (Vahl) Seigler & Ebinger Article History: subsp. detinens (Burch.) Kyal. & Boatwr., Boscia albitrunca (Burch.) Submission date: 25 Jun. 2019 Gilg & Gilg-Ben. var. albitrunca and Rhigozum trichotomum Burch. Revised: 14 Jan. 2020 The levels of Crude Protein (CP), Phosphorus (P), Calcium (C), Accepted: 16 Jan. 2020 Magnesium (Mg), Sodium (Na) and Potassium (K) were determined for Available online: 04 Apr. 2020 the plant’s foliage and pods (where available). All plant species had a https://bojaas.buan.ac.bw CP value higher than the recommended daily intake. There are however multiple mineral deficiencies in the plant species analysed. Nutritive Corresponding Author: value of Prosopis glandulosa is comparable to those other species despite the perception that livestock that browse on it are more Moffat P. -
Downloaded from Genbank (Refer to Table
COPYRIGHT AND CITATION CONSIDERATIONS FOR THIS THESIS/ DISSERTATION o Attribution — You must give appropriate credit, provide a link to the license, and indicate if changes were made. You may do so in any reasonable manner, but not in any way that suggests the licensor endorses you or your use. o NonCommercial — You may not use the material for commercial purposes. o ShareAlike — If you remix, transform, or build upon the material, you must distribute your contributions under the same license as the original. How to cite this thesis Surname, Initial(s). (2012) Title of the thesis or dissertation. PhD. (Chemistry)/ M.Sc. (Physics)/ M.A. (Philosophy)/M.Com. (Finance) etc. [Unpublished]: University of Johannesburg. Retrieved from: https://ujdigispace.uj.ac.za (Accessed: Date). ` The assessment of DNA barcoding as an identification tool for traded and protected trees in southern Africa: Mozambican commercial timber species as a case study By Ronny Mukala Kabongo Dissertation presented in the fulfilment of the requirements for the degree MAGISTER SCIENTIAE in BOTANY in the FACULTY OF SCIENCE DEPARTMENT OF BOTANY AND PLANT BIOTECHNOLOGY at the UNIVERSITY OF JOHANNESBURG SUPERVISOR: PROF MICHELLE VAN DER BANK CO-SUPERVISOR: DR. OLIVIER MAURIN January 2014 I hereby declare that this dissertation has been composed by me and work contained within unless stated otherwise, is my own. Signed: Ronny Mukala Kabongo Date: 30 January 2013 Table of Contents Table of Contents ......................................................................................................................... -
Akeake (Dodonaea Viscosa) Number 26
Akeake (Dodonaea viscosa) Number 26 DESCRIPTION Akeake is easily recognised by its distinctive bright green long thin wavy leaves, flaking bark and its papery winged seed capsules. It is a hardy, coastal shrub or small tree. In the right conditions, it will grow to about 10m high with a spreading crown and a trunk of around 30cm in diameter. The leaves are alternate, thin, light green with smooth margins and raised veins. The bark is reddish brown and peels off in long, thin strips. Akeake flowers are non-descript. They form in panicles at the end of branches from spring through to mid-summer. The fruit, however, are very visible as a capsule with two to four pale yellow wings which turn brown when dry and ripe. There are usually one or two seeds per capsule. DISTRIBUTION Akeake is native to New Zealand and grows throughout the North Island and northern parts of the South Island. It has also been found on the Chatham Islands, where it may have been introduced. It is also widely distributed through the Southern Hemisphere, with different subspecies and varieties in Australia, Southeast Asia, Africa and Latin CONSERVATION America. Akeake is not a nationally threatened or endangered plant. It is recognised as being Akeake naturally occurs in coastal and regionally distinctive, as it is generally lowland zones, favouring areas of open uncommon in the Taranaki region. forest or scrub. It prefers drier sites such as coastal river valleys, steep hillsides and THREATS cliffs and stabilised dunes. It is drought- Trampling and browsing by domestic tolerant and will handle light frosts. -
Dodonaea Viscosa Jacq. SAPINDACEAE Synonym: Dodonaea Angustifolia
Trees and Shrubs of the Maldives 83 Dodonaea viscosa Jacq. SAPINDACEAE Synonym: Dodonaea angustifolia . Common names: Giant bush hop, sand olive Dhivehi name: Kudhi-ruuvaali Status: Common in some of the islands in the southern group; rare in the northern islands. Description: A fast-growing small tree or shrub that grows to 1 to 3 m in height but is capable of reaching 8 m. Bark is dark grey coloured, fissured and peeling. Branchlets are rusty red in colour, sometimes narrowly winged or ridged. Branches exudate resin. Leaves are simple, papery with very short petiole or subsessile. Leaf shape varies from lanceolate to linear spooniform and secretes gummy exudate on both the surfaces and thus always appearing shiny. Leaf margin is entire or inconspicuously wavy and the leaf tip is pointed or round. Inflorescence is terminal or axillary and densely flowered. Flowers are very small, borne on long and slender flower stalks. Sepals are four in number, greenish-yellow in colour; petals are absent. Fruit is a capsule and two to three winged. In mature fruits, wings are coral red in colour. Seeds are small, black and lens like in shape. Each chamber of the fruit contains one to two seeds. Uses: D. viscosa is very effective in sand dune fixation and controlling coastal erosion since its roots are excellent soil binders. It is also used to reclaim marshes. It is grown as an ornamental plant for its shiny foliage and pink-red winged fruit. Poles are useful in fencing. Timber is hard and durable. In the Maldives, tree nails are prepared from the timber, which are used for boat building. -
Legumes of Wallace Desert Gardens
Bulletin of The Desert Legume Program of The Boyce Thompson Southwestern Arboretum and The University of Arizona Volume 18, Number 2 August 2006 Legumes of Wallace Desert Gardens Pamela Slate standing relationship between our Desert Gardens reviews and Botanic Coordinator organizations, one I see growing ever approves appropriate on-site Wallace Desert Gardens stronger year after year.” projects of mutual benefit. Wallace Desert Gardens is a Matthew B. Johnson non-profit foundation [(502(c)(3) In the mid-1980’s, the Program Manager and Curator under IRS rules] that was created in Wallace’s moved, complete with their Desert Legume Program 1993, well after much of the garden plant collection, from a Paradise was established. Its mission was Valley acre to a Scottsdale The virtues of desert legumes written by HB, as he was fondly subdivision where they purchased captured the attention of H.B. and known, to reflect the original intent of numerous acre-plus lots. At the time, Jocelyn M. Wallace when they first the foundation: HB had “no idea it would be bigger learned of the Desert Legume than a two-acre garden.” Although Program (DELEP) in 1989, about a Wallace Desert Gardens is a he “knew nothing of desert plants year after the program was founded collection of the world’s deserts when he moved to Arizona” in the at the University of Arizona. They plants located at an elevation of early 1980’s, they quickly became his understood the importance of some 2400 feet. Founded by passion. Today the garden legumes’ potential applications H.B. -
Die Plantfamilie ASTERACEAE: 6
ISSN 0254-3486 = SA Tydskrif vir Natuurwetenskap en Tegnologie 23, no. 1 & 2 2004 35 Algemene artikel Die plantfamilie ASTERACEAE: 6. Die subfamilie Asteroideae P.P.J. Herman Nasionale Botaniese Instituut, Privaat sak X101, Pretoria, 0001 e-pos: [email protected] UITTREKSEL Die tribusse van die subfamilie Asteroideae word meer volledig in hierdie artikel beskryf. Die genusse wat aan dié tribusse behoort word gelys en hulle verspreiding aangedui. ABSTRACT The plant family Asteraceae: 6. The subfamily Asteroideae. The tribes of the subfamily Asteroideae are described in this article. Genera belonging to the different tribes are listed and their distribution given. INLEIDING Tribus ANTHEMIDEAE Cass. Hierdie artikel is die laaste in die reeks oor die plantfamilie Verteenwoordigers van hierdie tribus is gewoonlik aromaties, Asteraceae.1-5 In die vorige artikel is die klassifikasie bokant byvoorbeeld Artemisia afra (wilde-als), Eriocephalus-soorte, familievlak asook die indeling van die familie Asteraceae in sub- Pentzia-soorte.4 Die feit dat hulle aromaties is, beteken dat hulle families en tribusse bespreek.5 Hierdie artikel handel oor die baie chemiese stowwe bevat. Hierdie stowwe word dikwels subfamilie Asteroideae van die familie Asteraceae, met ’n aangewend vir medisyne (Artemisia) of insekgif (Tanacetum).4 bespreking van die tribusse en die genusse wat aan die verskillende Verder is hulle blaartjies gewoonlik fyn verdeeld en selfs by dié tribusse behoort. Die ‘edelweiss’ wat in die musiekblyspel The met onverdeelde blaartjies, is die blaartjies klein en naaldvormig sound of music besing word, behoort aan die tribus Gnaphalieae (Erica-agtig). Die pappus bestaan gewoonlik uit vry of vergroeide van die subfamilie Asteroideae. -
Dodonaea Crucifolia (Sapindaceae, Dodonaeoideae), a New Species from North-Eastern New South Wales, Australia
Volume 23: 119–125 ELOPEA Publication date: 4 September 2020 T dx.doi.org/10.7751/telopea14438 Journal of Plant Systematics plantnet.rbgsyd.nsw.gov.au/Telopea • escholarship.usyd.edu.au/journals/index.php/TEL • ISSN 0312-9764 (Print) • ISSN 2200-4025 (Online) Dodonaea crucifolia (Sapindaceae, Dodonaeoideae), a new species from north-eastern New South Wales, Australia Ian R. H. Telford and Jeremy J. Bruhl Botany and N.C.W. Beadle Herbarium, School of Environmental and Rural Science, University of New England, Armidale, NSW 2351. Australia. Email: [email protected] Abstract Dodonaea crucifolia I.Telford & J.J.Bruhl (Sapindaceae, Dodonaeoideae), endemic to north-eastern New South Wales, Australia, and previously confused with D. hirsuta (Maiden & Betche) Maiden & Betche, is described as new. Dodonaea hirsuta is recircumscribed with the D. crucifolia components removed and with male flowers described for the first time. The distribution, habitat, and conservation status of both species are discussed and a table is provided comparing selected morphological attributes. Images of the new species and D. hirsuta are provided. The identification keys in Flora of Australia and NSW FloraOnline are modified to include the new species. Introduction During on-going investigations of taxa occurring on the New England Tableland of north-eastern New South Wales, Australia, and exhibiting disjunct patterns of distribution, differences became apparent between montane and coastal plants of Dodonaea hirsuta (Maiden & Betche) Maiden & Betche (Maiden and Betche 1902, 1913; Wilson and Scott 2002). Plants on the montane granite country at Torrington, New South Wales, and the Granite Belt, Queensland, and those from the coastal sandstones of the Grafton area, New South Wales exhibited differing attributes.