Formation of Nanoscale Th-Coffinite
Total Page:16
File Type:pdf, Size:1020Kb
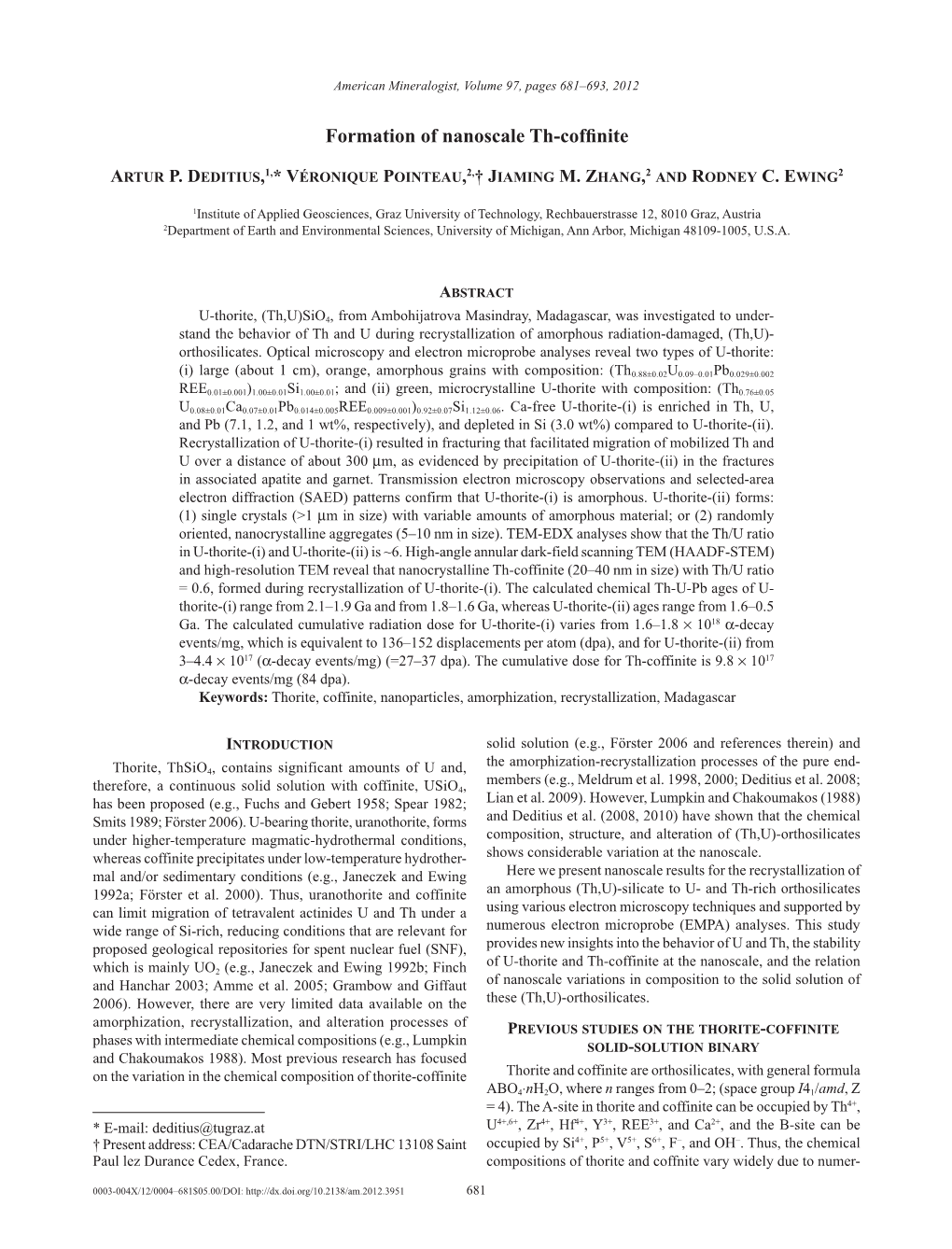
Load more
Recommended publications
-
Stillwellite-(Ce) (Ce, La, Ca)Bsio
Stillwellite-(Ce) (Ce; La; Ca)BSiO5 c 2001 Mineral Data Publishing, version 1.2 ° Crystal Data: Hexagonal. Point Group: 3: As °at rhombohedral crystals, to 4 cm, and massive. Twinning: Observed about [100]. Physical Properties: Cleavage: One imperfect. Fracture: Conchoidal. Hardness = 6.5 D(meas.) = 4.57{4.60 D(calc.) = 4.67 » Optical Properties: Transparent to translucent. Color: Red-brown to pale pink; colorless in thin section. Streak: White. Optical Class: Uniaxial (+) to biaxial (+). ! = 1.765{1.784 ² = 1.780{1.787 2V(meas.) = 0±{6± Cell Data: Space Group: P 31: a = 6.841{6.844 c = 6.700{6.702 Z = 3 X-ray Powder Pattern: Mary Kathleen mine, Australia. 3.43 (s), 2.96 (s), 2.13 (ms), 4.44 (m), 1.864 (m), 2.71 (mw), 2.24 (mw) Chemistry: (1) (2) (1) (2) (1) (2) SiO2 22.40 22.06 La2O3 27.95 19.12 MgO 0.06 UO2 0.22 Ce2O3 33.15 30.82 CaO 0.95 0.34 ThO2 5.41 Pr2O3 1.82 F 0.30 + B2O3 12.23 [13.46] Nd2O3 5.36 H2O 0.85 Al2O3 0.42 Sm2O3 0.34 H2O¡ 0.10 Y2O3 0.74 0.28 Fe2O3 0.18 P2O5 0.67 Total [100.00] [99.26] (1) Mary Kathleen mine, Australia; recalculated to 100.00% after removal of very small amounts of uraninite and apatite determined by separate analysis. (2) Vico volcano, near Vetralla, Italy; by electron microprobe, B2O3 calculated from stoichiometry, original total given as 99.23%; corresponds to (Ce0:50La0:31Nd0:08Th0:05Pr0:03Ca0:02Sm0:01)§=1:00B1:02Si0:97O5: Occurrence: Locally abundant as a metasomatic replacement of metamorphosed calcareous sediments (Mary Kathleen mine, Australia); in alkalic pegmatites in syenite in an alkalic massif (Dara-i-Pioz massif, Tajikistan). -
Identification and Occurrence of Uranium and Vanadium Minerals from the Colorado Plateaus
SpColl £2' 1 Energy I TEl 334 Identification and Occurrence of Uranium and Vanadium Minerals from the Colorado Plateaus ~ By A. D. Weeks and M. E. Thompson ~ I"\ ~ ~ Trace Elements Investigations Report 334 UNITED STATES DEPARTMENT OF THE INTERIOR GEOLOGICAL SURVEY IN REPLY REFER TO: UNITED STATES DEPARTMENT OF THE INTERIOR GEOLOGICAL SURVEY WASHINGTON 25, D. C. AUG 12 1953 Dr. PhilUp L. Merritt, Assistant Director Division of Ra1'r Materials U. S. AtoTILic Energy Commission. P. 0. Box 30, Ansonia Station New· York 23, Nei< York Dear Phil~ Transmitted herewith are six copies oi' TEI-334, "Identification and occurrence oi' uranium and vanadium minerals i'rom the Colorado Plateaus," by A , D. Weeks and M. E. Thompson, April 1953 • We are asking !41'. Hosted to approve our plan to publish this re:por t as a C.i.rcular .. Sincerely yours, Ak~f777.~ W. H. ~radley Chief' Geologist UNCLASSIFIED Geology and Mineralogy This document consists or 69 pages. Series A. UNITED STATES DEPARTMENT OF TEE INTERIOR GEOLOGICAL SURVEY IDENTIFICATION AND OCCURRENCE OF URANIUM AND VANADIUM MINERALS FROM TEE COLORADO PLATEAUS* By A• D. Weeks and M. E. Thompson April 1953 Trace Elements Investigations Report 334 This preliminary report is distributed without editorial and technical review for conformity with ofricial standards and nomenclature. It is not for public inspection or guotation. *This report concerns work done on behalf of the Division of Raw Materials of the u. s. Atomic Energy Commission 2 USGS GEOLOGY AllU MINEFALOGY Distribution (Series A) No. of copies American Cyanamid Company, Winchester 1 Argulllle National La:boratory ., ., ....... -
Three Occurrences of High-Thorian Uraninite Near Easton, Pennsylvania*
THE AMERICAN MINERALOGIST, YOL. 42, NOVEMBER_DECEMBER 1957 THREE OCCURRENCES OF HIGH-THORIAN URANINITE NEAR EASTON, PENNSYLVANIA* Anruun M oNrcounnu, Laf ayette C oll,ege, Easton, P ennsylaanio. Assrnacr The geology, mineralogy and paragenesis of three occurrences of high-thorian uraninite in serpentine near Easton, Pa., are described. seven *-ray fluorescence analyses together with three corroborative chemical analyses show this mineral to be high-thorian uraninite rather than high-uranoan thorianite as supposed six samples contajn from about l5/o to 3570ThOr. At the Williams quarry hydrothermai alteration of much uraninite to a sequence of secondary uranium- and thorium-rich minerals occurred. Frondel,s Mineral C, thoro- gummite, then boltrvoodite and uranophane were formed during serpentinization of frac- tured dolomite-diopside-tremolite contact-metamorphosed rock. Supergene coatings, chiefly hydrous uranium silicates and including boltwoodite and uranophane, formed much later. At the reservoir site slight hldrothermal alteration oI uraninite to thorogummite occurred. At the Royal Green Marble co. quarry uraninite is found unaltered. At these localities fracturing or temperatures during later hydrothermal mineralization may have been of lower intensity. It is concluded that thorium-rich Easton uraninite does not alter readily to secondary minerals, except when subjected to severe deformation and concomitant attack by ser- pentinizing hydrothermal solutions. The Th, rJ and. zr of this contact-metasomatic uran- inite and the associated zircon originated in a granite-pegmatite magma rich in these elements, which intruded dolomitic rocks and through deformation while crystallizing yielded the hydrothermal solutions that deposited these minerals in those rocks. then serpentinized the rocks. INrnonucrroN High-thorian uraninite, regardeduntil now as high-uranoan thorian- ite,1occurs in serpentinerock at three localitiesjust north and northeast of Easton, Pennsylvania.The two earlier discoverieswere made around 1930by GeorgeW. -
Technical Report 89-17
Nagra Cedra Cisra Nationale Societe cooperative Societe. cooperativa Genossenschaft nationale nazionale fUr die Lagerung pour I'entreposage per I'immagazzinamento radioaktiver Abfalle de dechets radioactifs di scorie radioattive TECHNICAL REPORT 89-17 REDUCTION SPHERES IN HEMATITIC ROCKS FROM NORTHERN SWITZERLAND: IMPLICATIONS FOR THE MOBILITY OF SOME RARE ELEMENTS BEDA A. HOFMANN NOVEMBER 1990 Mineralogisch-petrographisches Institut der UniversiUit Bern Present address: Naturhistorisches Museum, Bernastr. 15,3005 Bern Parkstrasse 23 5401 Baden 1 Schweiz Telephon 056/205511 Nagra Cedra Cisra Nationale Societe cooperative Societe. cooperativa Genossenschaft nationale nazionale fUr die Lagerung pour I'entreposage per I'immagazzinamento radioaktiver Abfalle de dechets radioactifs di scorie radioattive TECHNICAL REPORT 89-17 REDUCTION SPHERES IN HEMATITIC ROCKS FROM NORTHERN SWITZERLAND: IMPLICATIONS FOR THE MOBILITY OF SOME RARE ELEMENTS BEDA A. HOFMANN NOVEMBER 1990 Mineralogisch-petrographisches Institut der UniversiUit Bern Present address: Naturhistorisches Museum, Bernastr. 15,3005 Bern Parkstrasse 23 5401 Baden 1 Schweiz Telephon 056/205511 This report was prepared as an account of work sponsored by Nagra. The viewpoints presented and conclusions reached are those of the author and do not necessarily represent those of Nagra. "Copyright (c) 1991 by Nagra, Baden (Switzerland). / All rights reserved. A11 parts of th i s work are protected by copyr i ght. Any util i sat i on outwi th the remit of the copyright law is unlawful and liable to prosecution. This applies in particular to trans'lations, storage and processing in electronic systems and programs, microfilms, reproductions, etc." NAGRA NTB 89-17 - I - ABSTRACT Reduction spheres are small-scale, isolated redox systems occurring in hematite-stained rocks of variable age, origin and provenience. -
Petrology and Mineralogy of the Mount Rosa Area, El Paso and Teller Counties, Colorado
THE AMERICAN MINERALOGIST, VOL. 5 1, MARCH-APRIL, 1966 PETROLOGY AND MINERALOGY OF THE MOUNT ROSA AREA, EL PASO AND TELLER COUNTIES, COLORADO. II. PEGMATITESI EuceNB B. Gnoss, CaliJornia Diris,ion of Mines and Geology San Fra nci sco, C alifor n i o AND E. Wu. IIerNnrcu, Department of Geologyand Mineralogy The Llniversity of Michigan, Ann Arbor, Michigan. Aesrnlcr Two contrasting swarms of pegmatite dikes occur intermingled in the Pikes Peak granite and in related alkalic granites in the Mount Rosa area, west of Colorado Springs. One is the older calc-alkalic Pikes Peak type; the younger is the alkalic Mount Rosa type. The accessory assemblage of the Mount Rosa quartz-microcline-riebeckite pegmatites consists chiefly of radioactive zircon, fluorite, astrophyllite, thorite, and lesser amounts of pyrochlore, rutile, niobian rutile, columbite, bertrandite and rare aluminofluorides. These dikes intrude both the Pikes Peak and its derivative fayaiite granites along north- east-trending fractures. These pegmatites contrast markedly with the coexisting Pikes Peak pegmatites, which are characterized by quartz and amazonite crystals, zircon, biotite, siderite, and minor topaz, allanite, cassiterite and genthelvite. The Pikes Peak type occurs as segregations and injected bodies only within Pikes Peak granite. Age determinations on zircons from pegmatites of the Mount Rosa type proved to be inconsistent, but ages determined by means of the Kao/Ara0 method on pegmatitic rie- beckite are consistent rvith geological evidence and age determinations on the granites, giving an age of 1040 my. INrnolucrroN Discoverl' of unusual minerals in pegmatitesof the St. Peters Dome district, Mount Rosa area,Colorado, attracted the attention of mineralo- gists as earlv as 1877 (Koenig, 1877). -
The Uranium-Vanadium Ore Deposit at the Monument No. 1-Mitten No. 2 Mine, Monument Valley Navajo County, Arizona
The Uranium-Vanadium Ore Deposit at the Monument No. 1-Mitten No. 2 Mine, Monument Valley Navajo County, Arizona GEOLOGICAL SURVEY BULLETIN 1107-C Prepared on behalf of the U. S. Atomic Energy Commission and published with the permission of the Commission The Uranium-Vanadium Ore Deposit at the Monument No. 1-Mitten No. 2 Mine, Monument Valley Navajo County, Arizona By IRVING J. WITKIND CONTRIBUTIONS TO THE GEOLOGY OF URANIUM GEOLOGICAL SURVEY BULLETIN 1107-C Prepared on behalf of the U.S. Atomic Energy Commission and published with the permission of the Commission UNITED STATES GOVERNMENT PRINTING OFFICE, WASHINGTON : 1961 UNITED STATES DEPARTMENT OF THE INTERIOR STEWART L. UDALL, Secretary GEOLOGICAL SURVEY Thomas B. Nolan, Director For sale by the Superintendent of Documents, U.S. Government Printing Office Washington 25, D.C. CONTENTS Paga Abstract.________________________________________________________ 219 Introduction._ ____________________________________________________ 219 Purpose of work_______________________________________________ 221 Location of the mine___________________________________________ 221 History of mining. ____________________________________________ 221 Previous work_________________________________________________ 223 Acknowledgments _____________________________________________ 223 Geology_--___________-.__________________________________________ 224 General._____________________________________________________ 224 Moenkopi formation ___________________________________________ 225 Chinle formation. _____________________________________________ -
Thermodynamics of Formation of Coffinite, Usio4
Thermodynamics of formation of coffinite, USiO4 Xiaofeng Guoa,b, Stéphanie Szenknectc, Adel Mesbahc, Sabrina Labsd, Nicolas Clavierc, Christophe Poinssote, Sergey V. Ushakova, Hildegard Curtiusd, Dirk Bosbachd, Rodney C. Ewingf, Peter C. Burnsg, Nicolas Dacheuxc, and Alexandra Navrotskya,1 aPeter A. Rock Thermochemistry Laboratory and Nanomaterials in the Environment, Agriculture, and Technology Organized Research Unit, University of California, Davis, CA 95616; bEarth and Environmental Sciences Division, Los Alamos National Laboratory, Los Alamos, NM 87545; cInstitut de Chimie Séparative de Marcoule, UMR 5257, CNRS/CEA/Université Montpellier/Ecole Nationale Supérieure de Chimie de Montpellier, Site de Marcoule, 30207 Bagnols sur Cèze, France; dInstitute of Energy and Climate Research, Nuclear Waste Management, Forschungszentrum Jülich GmbH, 52425 Jülich, Germany; eCEA, Nuclear Energy Division, RadioChemistry & Processes Department, BP 17171, 30207 Bagnols sur Cèze, France; fDepartment of Geological and Environmental Sciences, School of Earth Sciences, Stanford University, Stanford, CA 94305; and gDepartment of Civil and Environmental Engineering and Earth Sciences, University of Notre Dame, Notre Dame, IN 46556 Contributed by Alexandra Navrotsky, April 20, 2015 (sent for review February 10, 2015) Coffinite, USiO4, is an important U(IV) mineral, but its thermody- The precipitation of USiO4 as a secondary phase should be namic properties are not well-constrained. In this work, two dif- favored in contact with silica-rich groundwater (21) [silica − ferent coffinite samples were synthesized under hydrothermal concentration >10 4 mol/L (22, 23)]. Natural coffinite samples conditions and purified from a mixture of products. The enthalpy are often fine-grained (4, 5, 8, 11, 13, 15, 24), due to the long of formation was obtained by high-temperature oxide melt solu- exposure to alpha-decay event irradiation (4, 6, 25, 26) and are tion calorimetry. -
Primary Minerals of the Jáchymov Ore District
Journal of the Czech Geological Society 48/34(2003) 19 Primary minerals of the Jáchymov ore district Primární minerály jáchymovského rudního revíru (237 figs, 160 tabs) PETR ONDRU1 FRANTIEK VESELOVSKÝ1 ANANDA GABAOVÁ1 JAN HLOUEK2 VLADIMÍR REIN3 IVAN VAVØÍN1 ROMAN SKÁLA1 JIØÍ SEJKORA4 MILAN DRÁBEK1 1 Czech Geological Survey, Klárov 3, CZ-118 21 Prague 1 2 U Roháèových kasáren 24, CZ-100 00 Prague 10 3 Institute of Rock Structure and Mechanics, V Holeovièkách 41, CZ-182 09, Prague 8 4 National Museum, Václavské námìstí 68, CZ-115 79, Prague 1 One hundred and seventeen primary mineral species are described and/or referenced. Approximately seventy primary minerals were known from the district before the present study. All known reliable data on the individual minerals from Jáchymov are presented. New and more complete X-ray powder diffraction data for argentopyrite, sternbergite, and an unusual (Co,Fe)-rammelsbergite are presented. The follow- ing chapters describe some unknown minerals, erroneously quoted minerals and imperfectly identified minerals. The present work increases the number of all identified, described and/or referenced minerals in the Jáchymov ore district to 384. Key words: primary minerals, XRD, microprobe, unit-cell parameters, Jáchymov. History of mineralogical research of the Jáchymov Chemical analyses ore district Polished sections were first studied under the micro- A systematic study of Jáchymov minerals commenced scope for the identification of minerals and definition early after World War II, during the period of 19471950. of their relations. Suitable sections were selected for This work was aimed at supporting uranium exploitation. electron microprobe (EMP) study and analyses, and in- However, due to the general political situation and the teresting domains were marked. -
Trace- and Minor-Element Mineralogy of the Microgranite at Ghurayyah
DEPARTMENT OF THE INTERIOR U.S. GEOLOGICAL SURVEY Trace- and minor-element mineralogy of the microgranite at Ghurayyah, Kingdom of Saudi Arabia I/ I/ Mortimer H. Staatz and Isabelle K. Brownfield Open-File Report 86- Report prepared for Ministry of Petroleum and Mineral Resources, Deputy Ministry for Mineral Resources, Saudi Arabia This report is preliminary and has not been reviewed for conformity with U.S. Geological Survey editorial standards and stratigraphic nomenclature. I/ U.S. Geological Survey, Denver, CO 1986 CONTENTS ABSTRACT........................................................... 1 INTRODUCTION....................................................... 1 MINERAL-SEPARATION STUDIES......................................... 4 AUTORADIOGRAPHS.................................................... 6 INDIVIDUAL-GRAIN STUDIES........................................... 6 GRAIN-SIZE STUDIES................................................. 12 DATA STORAGE....................................................... 12 REFERENCES CITED................................................... 12 ILLUSTRATIONS Figure 1 Index map showing the location of Ghurayyah.............. 2 2 Autoradiographs of samples from Ghurayyah................ 7 TABLES Table 1 Location and type of samples collected at Ghurayyah....... 3 2 Minerals identified in heavy-mineral separates from Ghurayyah................................................. 5 3 Electron-microprobe analyses of zircon from Ghurayyah..... 9 4 Electron-microprobe analyses of thorite grains from sample G-l, -
Coffinite Formation from UO2+X
www.nature.com/scientificreports OPEN Cofnite formation from UO2+x Stéphanie Szenknect1*, Delhia Alby1, Marta López García2, Chenxu Wang4, Renaud Podor1, Frédéric Miserque5, Adel Mesbah1, Lara Duro2, Lena Zetterström Evins3, Nicolas Dacheux1, Jordi Bruno2 & Rodney C. Ewing4 Most of the highly radioactive spent nuclear fuel (SNF) around the world is destined for fnal disposal in deep-mined geological repositories. At the end of the fuel’s useful life in a reactor, about 96% of the SNF is still UO2. Thus, the behaviour of UO2 in SNF must be understood and evaluated under the weathering conditions of geologic disposal, which extend to periods of hundreds of thousands of years. There is ample evidence from nature that many uranium deposits have experienced conditions for which the formation of cofnite, USiO4, has been favoured over uraninite, UO2+x, during subsequent alteration events. Thus, cofnite is an important alteration product of the UO2 in SNF. Here, we present the frst evidence of the formation of cofnite on the surface of UO2 at the time scale of laboratory experiments in a solution saturated with respect to amorphous silica at pH = 9, room temperature and under anoxic conditions. 4+ Uraninite, UO2+x is the most common U mineral in nature followed by cofnite, USiO 4, which is found as a primary phase or an alteration product in many uranium deposits. Cofnite, tetragonal, is isostructural with 1 zircon (ZrSiO4) and thorite (TSiO 4); however, cofnite can contain some water either as H 2O or OH groups . Altered uraninite and cofnite have been documented from Oklo, Gabon 2–5, deposits in the Athabasca Basin 4,6,7 and Elliot Lake, Canada8. -
Diagenetic Formation of Uranium-Silica Polymers in Lake Sediments Over 3,300 Years
Diagenetic formation of uranium-silica polymers in lake sediments over 3,300 years Pierre Lefebvrea,1, Alkiviadis Gourgiotisb, Arnaud Mangeretb, Pierre Sabatierc, Pierre Le Papea, Olivier Diezb, Pascale Louvatd, Nicolas Menguya, Pauline Merrota,2, Camille Bayaa, Mathilde Zebrackib, Pascale Blanchartb, Emmanuel Maletc, Didier Jézéqueld,e, Jean-Louis Reyssc, John R. Bargarf, Jérôme Gaillardetd, Charlotte Cazalab, and Guillaume Morina aInstitut de Minéralogie, de Physique des Matériaux et de Cosmochimie, UMR 7590 Sorbonne Université-CNRS-MNHN-IRD (Museum National d’Histoire Naturelle-Institut de Recherche pour le Développement), 75005 Paris, France; bInstitut de Radioprotection et de Sûreté Nucléaire, PSE-ENV SEDRE, 92260 Fontenay-aux-Roses, France; cUMR 5204, Université Grenoble Alpes-Université Savoie Mont Blanc-CNRS-EDYTEM (Environnements DYnamiques et TErritoires de la Montagne), 73370 Le Bourget-Du-Lac, France; dUMR 7154, Université de Paris-Institut de Physique du Globe de Paris-CNRS, 75005 Paris, France; eINRAE-Université Savoie Mont Blanc, UMR CARRTEL (Centre Alpin de Recherche sur les Réseaux Trophiques et Ecosystèmes Limniques), 74200 Thonon-les-Bains, France; and fStanford Synchrotron Radiation Lightsource, SLAC National Accelerator Laboratory, Menlo Park, CA 94025 Edited by François M. M. Morel, Princeton University, Princeton, NJ, and approved December 16, 2020 (received for review October 27, 2020) The long-term fate of uranium-contaminated sediments, especially have been recognized as a major U pool in reduced contami- downstream former mining areas, is a widespread environmental nated soils and sediments over the last decade (6, 7, 19–25) and challenge. Essential for their management is the proper under- are known to be sensitive to reoxidation by natural oxidants such standing of uranium (U) immobilization mechanisms in reducing as oxygen or nitrate (26 and references therein). -
Report 93-17
REPORT 93-17 Oxidation of uraninite Janusz Janeczek, Rodney C Ewing Department of Earth & Planetary Science, University of New Mexico, Albuquerque, NM, USA June 1993 SVENSK KARNBRANSLEHANTERING AB SWEDISH NUCLEAR FUEL AND WASTE MANAGEMENT CO BOX 5864 S-102 48 STOCKHOLM TEL 08-665 28 00 TELEX 13108 SKB S TELEFAX 08-661 57 19 OXIDATION OF URANINITE Janusz Janeczek, Rodney C Ewing Department of Earth & Planetary Science, University of New Mexico, Albuquerque, NH, USA June 1993 This report concerns a study which was conducted for SKB. The conclusions and viewpoints presented in the report are those of the author(s) and do not necessarily coincide with those of the client. Information on SKB technical reports from 1977-1978 (TR 121), 1979 (TR 79-28), 1980 (TR 80-26), 1981 (TR 81-17), 1982 (TR 82-28), 1983 (TR 83-77), 1984 (TR 85-01), 1985 (TR 85-20), 1986 (TR 86-31), 1987 (TR 87-33), 1988 (TR 88-32), -1989 (TR 89-40), 1990 (TR 90-46) and 1991 (TR 91-64) is available through SKB. Oxidation of Uraninite Janusz Janeczek* Rodney C. Ewing Department of Earth & Planetary Sciences, University of New Mexico Albuquerque, New Mexico 87131, U.S.A. •presently at Departure Jt of Earth Sciences, Silesian University, Bedr i ska 60,41-200 Sosnowiec, Poland June, 1993 Keywords: spent nuclear fuel, uraninite, UO2, oxidation, corrosion, natural analogue, Cigar Lake. ABSTRACT Samples of uraninite and pitchblende annealed at 1200°C in H2, and untreated pitchblende were sequentially oxidized in air at 18O-19O°C, 230°C, and 300°C.