Lecture 12: Phonon Heat Capacity
Total Page:16
File Type:pdf, Size:1020Kb
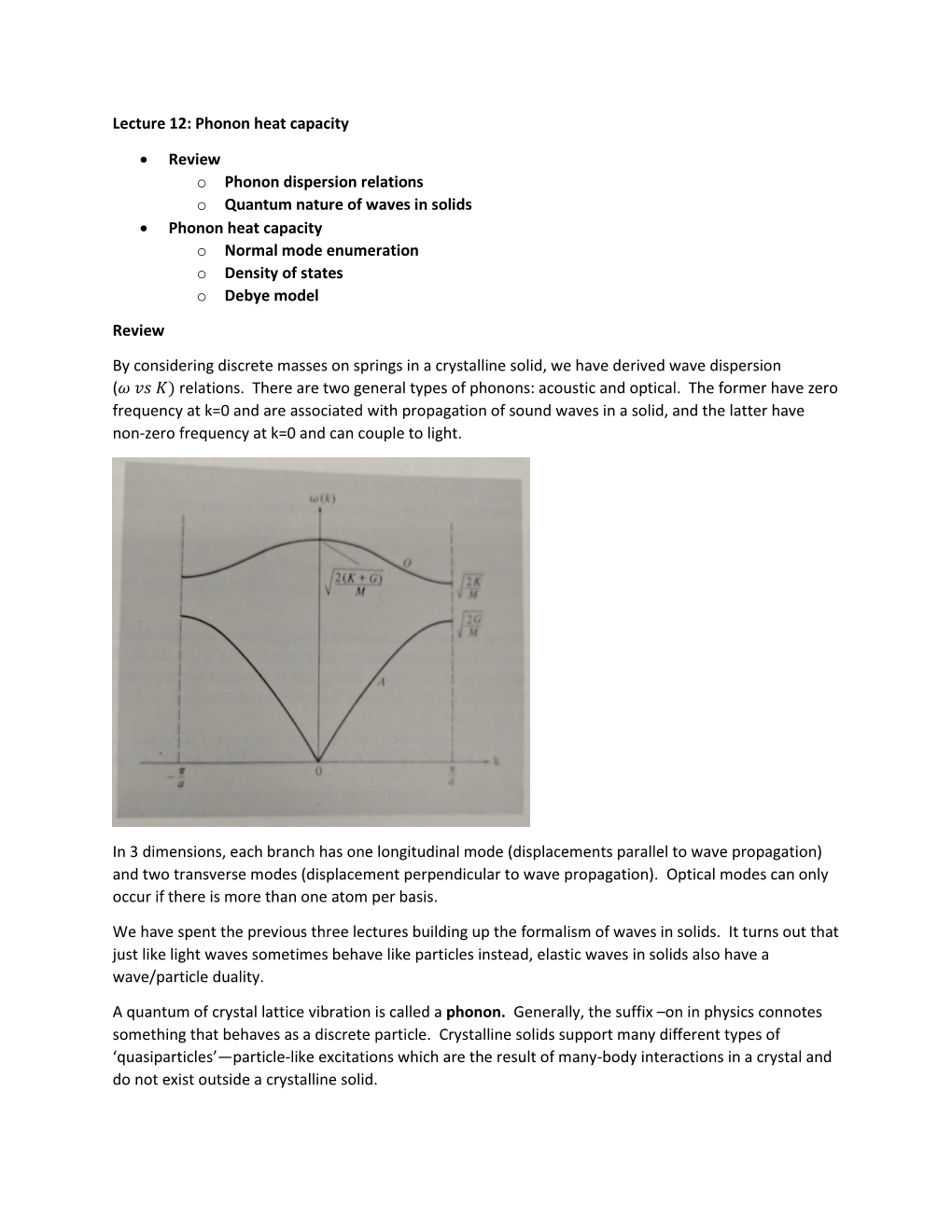
Load more
Recommended publications
-
Part 5: the Bose-Einstein Distribution
PHYS393 – Statistical Physics Part 5: The Bose-Einstein Distribution Distinguishable and indistinguishable particles In the previous parts of this course, we derived the Boltzmann distribution, which described how the number of distinguishable particles in different energy states varied with the energy of those states, at different temperatures: N εj nj = e− kT . (1) Z However, in systems consisting of collections of identical fermions or identical bosons, the wave function of the system has to be either antisymmetric (for fermions) or symmetric (for bosons) under interchange of any two particles. With the allowed wave functions, it is no longer possible to identify a particular particle with a particular energy state. Instead, all the particles are “shared” between the occupied states. The particles are said to be indistinguishable . Statistical Physics 1 Part 5: The Bose-Einstein Distribution Indistinguishable fermions In the case of indistinguishable fermions, the wave function for the overall system must be antisymmetric under the interchange of any two particles. One consequence of this is the Pauli exclusion principle: any given state can be occupied by at most one particle (though we can’t say which particle!) For example, for a system of two fermions, a possible wave function might be: 1 ψ(x1, x 2) = [ψ (x1)ψ (x2) ψ (x2)ψ (x1)] . (2) √2 A B − A B Here, x1 and x2 are the coordinates of the two particles, and A and B are the two occupied states. If we try to put the two particles into the same state, then the wave function vanishes. Statistical Physics 2 Part 5: The Bose-Einstein Distribution Indistinguishable fermions Finding the distribution with the maximum number of microstates for a system of identical fermions leads to the Fermi-Dirac distribution: g n = i . -
The Debye Model of Lattice Heat Capacity
The Debye Model of Lattice Heat Capacity The Debye model of lattice heat capacity is more involved than the relatively simple Einstein model, but it does keep the same basic idea: the internal energy depends on the energy per phonon (ε=ℏΩ) times the ℏΩ/kT average number of phonons (Planck distribution: navg=1/[e -1]) times the number of modes. It is in the number of modes that the difference between the two models occurs. In the Einstein model, we simply assumed that each mode was the same (same frequency, Ω), and that the number of modes was equal to the number of atoms in the lattice. In the Debye model, we assume that each mode has its own K (that is, has its own λ). Since Ω is related to K (by the dispersion relation), each mode has a different Ω. 1. Number of modes In the Debye model we assume that the normal modes consist of STANDING WAVES. If we have travelling waves, they will carry energy through the material; if they contain the heat energy (that will not move) they need to be standing waves. Standing waves are waves that do travel but go back and forth and interfere with each other to create standing waves. Recall that Newton's Second Law gave us atoms that oscillate: [here x = sa where s is an integer and a the lattice spacing] iΩt iKsa us = uo e e ; and if we use the form sin(Ksa) for eiKsa [both indicate oscillations]: iΩt us = uo e sin(Ksa) . We now apply the boundary conditions on our solution: to have standing waves both ends of the wave must be tied down, that is, we must have u0 = 0 and uN = 0. -
Phys 446: Solid State Physics / Optical Properties Lattice Vibrations
Solid State Physics Lecture 5 Last week: Phys 446: (Ch. 3) • Phonons Solid State Physics / Optical Properties • Today: Einstein and Debye models for thermal capacity Lattice vibrations: Thermal conductivity Thermal, acoustic, and optical properties HW2 discussion Fall 2007 Lecture 5 Andrei Sirenko, NJIT 1 2 Material to be included in the test •Factors affecting the diffraction amplitude: Oct. 12th 2007 Atomic scattering factor (form factor): f = n(r)ei∆k⋅rl d 3r reflects distribution of electronic cloud. a ∫ r • Crystalline structures. 0 sin()∆k ⋅r In case of spherical distribution f = 4πr 2n(r) dr 7 crystal systems and 14 Bravais lattices a ∫ n 0 ∆k ⋅r • Crystallographic directions dhkl = 2 2 2 1 2 ⎛ h k l ⎞ 2πi(hu j +kv j +lw j ) and Miller indices ⎜ + + ⎟ •Structure factor F = f e ⎜ a2 b2 c2 ⎟ ∑ aj ⎝ ⎠ j • Definition of reciprocal lattice vectors: •Elastic stiffness and compliance. Strain and stress: definitions and relation between them in a linear regime (Hooke's law): σ ij = ∑Cijklε kl ε ij = ∑ Sijklσ kl • What is Brillouin zone kl kl 2 2 C •Elastic wave equation: ∂ u C ∂ u eff • Bragg formula: 2d·sinθ = mλ ; ∆k = G = eff x sound velocity v = ∂t 2 ρ ∂x2 ρ 3 4 • Lattice vibrations: acoustic and optical branches Summary of the Last Lecture In three-dimensional lattice with s atoms per unit cell there are Elastic properties – crystal is considered as continuous anisotropic 3s phonon branches: 3 acoustic, 3s - 3 optical medium • Phonon - the quantum of lattice vibration. Elastic stiffness and compliance tensors relate the strain and the Energy ħω; momentum ħq stress in a linear region (small displacements, harmonic potential) • Concept of the phonon density of states Hooke's law: σ ij = ∑Cijklε kl ε ij = ∑ Sijklσ kl • Einstein and Debye models for lattice heat capacity. -
A Short Review of Phonon Physics Frijia Mortuza
International Journal of Scientific & Engineering Research Volume 11, Issue 10, October-2020 847 ISSN 2229-5518 A Short Review of Phonon Physics Frijia Mortuza Abstract— In this article the phonon physics has been summarized shortly based on different articles. As the field of phonon physics is already far ad- vanced so some salient features are shortly reviewed such as generation of phonon, uses and importance of phonon physics. Index Terms— Collective Excitation, Phonon Physics, Pseudopotential Theory, MD simulation, First principle method. —————————— —————————— 1. INTRODUCTION There is a collective excitation in periodic elastic arrangements of atoms or molecules. Melting transition crystal turns into liq- uid and it loses long range transitional order and liquid appears to be disordered from crystalline state. Collective dynamics dispersion in transition materials is mostly studied with a view to existing collective modes of motions, which include longitu- dinal and transverse modes of vibrational motions of the constituent atoms. The dispersion exhibits the existence of collective motions of atoms. This has led us to undertake the study of dynamics properties of different transitional metals. However, this collective excitation is known as phonon. In this article phonon physics is shortly reviewed. 2. GENERATION AND PROPERTIES OF PHONON Generally, over some mean positions the atoms in the crystal tries to vibrate. Even in a perfect crystal maximum amount of pho- nons are unstable. As they are unstable after some time of period they come to on the object surface and enters into a sensor. It can produce a signal and finally it leaves the target object. In other word, each atom is coupled with the neighboring atoms and makes vibration and as a result phonon can be found [1]. -
Intermediate Statistics in Thermoelectric Properties of Solids
Intermediate statistics in thermoelectric properties of solids André A. Marinho1, Francisco A. Brito1,2 1 Departamento de Física, Universidade Federal de Campina Grande, 58109-970 Campina Grande, Paraíba, Brazil and 2 Departamento de Física, Universidade Federal da Paraíba, Caixa Postal 5008, 58051-970 João Pessoa, Paraíba, Brazil (Dated: July 23, 2019) Abstract We study the thermodynamics of a crystalline solid by applying intermediate statistics manifested by q-deformation. We based part of our study on both Einstein and Debye models, exploring primarily de- formed thermal and electrical conductivities as a function of the deformed Debye specific heat. The results revealed that the q-deformation acts in two different ways but not necessarily as independent mechanisms. It acts as a factor of disorder or impurity, modifying the characteristics of a crystalline structure, which are phenomena described by q-bosons, and also as a manifestation of intermediate statistics, the B-anyons (or B-type systems). For the latter case, we have identified the Schottky effect, normally associated with high-Tc superconductors in the presence of rare-earth-ion impurities, and also the increasing of the specific heat of the solids beyond the Dulong-Petit limit at high temperature, usually related to anharmonicity of interatomic interactions. Alternatively, since in the q-bosons the statistics are in principle maintained the effect of the deformation acts more slowly due to a small change in the crystal lattice. On the other hand, B-anyons that belong to modified statistics are more sensitive to the deformation. PACS numbers: 02.20-Uw, 05.30-d, 75.20-g arXiv:1907.09055v1 [cond-mat.stat-mech] 21 Jul 2019 1 I. -
Quantum Phonon Optics: Coherent and Squeezed Atomic Displacements
PHYSICAL REVIEW B VOLUME 53, NUMBER 5 1 FEBRUARY 1996-I Quantum phonon optics: Coherent and squeezed atomic displacements Xuedong Hu and Franco Nori Department of Physics, The University of Michigan, Ann Arbor, Michigan 48109-1120 ~Received 17 August 1995; revised manuscript received 27 September 1995! We investigate coherent and squeezed quantum states of phonons. The latter allow the possibility of modu- lating the quantum fluctuations of atomic displacements below the zero-point quantum noise level of coherent states. The expectation values and quantum fluctuations of both the atomic displacement and the lattice amplitude operators are calculated in these states—in some cases analytically. We also study the possibility of squeezing quantum noise in the atomic displacement using a polariton-based approach. I. INTRODUCTION words, a coherent state is as ‘‘quiet’’ as the vacuum state. Squeezed states5 are interesting because they can have Classical phonon optics1 has succeeded in producing smaller quantum noise than the vacuum state in one of the many acoustic analogs of classical optics, such as phonon conjugate variables, thus having a promising future in differ- mirrors, phonon lenses, phonon filters, and even ‘‘phonon ent applications ranging from gravitational wave detection to microscopes’’ that can generate acoustic pictures with a reso- optical communications. In addition, squeezed states form an lution comparable to that of visible light microscopy. Most exciting group of states and can provide unique insight into phonon optics experiments use heat pulses or superconduct- quantum mechanical fluctuations. Indeed, squeezed states are ing transducers to generate incoherent phonons, which now being explored in a variety of non-quantum-optics sys- 6 propagate ballistically in the crystal. -
Arxiv:1608.06287V1 [Hep-Th] 22 Aug 2016 Published in Int
Preprint typeset in JHEP style - HYPER VERSION Surprises with Nonrelativistic Naturalness Petr Hoˇrava Berkeley Center for Theoretical Physics and Department of Physics University of California, Berkeley, CA 94720-7300, USA and Theoretical Physics Group, Lawrence Berkeley National Laboratory Berkeley, CA 94720-8162, USA Abstract: We explore the landscape of technical naturalness for nonrelativistic systems, finding surprises which challenge and enrich our relativistic intuition already in the simplest case of a single scalar field. While the immediate applications are expected in condensed matter and perhaps in cosmology, the study is motivated by the leading puzzles of funda- mental physics involving gravity: The cosmological constant problem and the Higgs mass hierarchy problem. This brief review is based on talks and lectures given at the 2nd LeCosPA Symposium on Everything About Gravity at National Taiwan University, Taipei, Taiwan (December 2015), to appear in the Proceedings; at the International Conference on Gravitation and Cosmology, KITPC, Chinese Academy of Sciences, Beijing, China (May 2015); at the Symposium Celebrating 100 Years of General Relativity, Guanajuato, Mexico (November 2015); and at the 54. Internationale Universit¨atswochen f¨urTheoretische Physik, Schladming, Austria (February 2016). arXiv:1608.06287v1 [hep-th] 22 Aug 2016 Published in Int. J. Mod. Phys. D Contents 1. Puzzles of Naturalness 1 1.1 Technical Naturalness 2 2. Naturalness in Nonrelativistic Systems 3 2.1 Towards the Classification of Nonrelativistic Nambu-Goldstone Modes 3 2.2 Polynomial Shift Symmetries 5 2.3 Naturalness of Cascading Hierarchies 5 3. Interacting Theories with Polynomial Shift Symmetries 6 3.1 Invariants of Polynomial Shift Symmetries 6 3.2 Polynomial Shift Invariants and Graph Theory 6 3.3 Examples 7 4. -
Quantum Phases and Spin Liquid Properties of 1T-Tas2 ✉ Samuel Mañas-Valero 1, Benjamin M
www.nature.com/npjquantmats ARTICLE OPEN Quantum phases and spin liquid properties of 1T-TaS2 ✉ Samuel Mañas-Valero 1, Benjamin M. Huddart 2, Tom Lancaster 2, Eugenio Coronado1 and Francis L. Pratt 3 Quantum materials exhibiting magnetic frustration are connected to diverse phenomena, including high Tc superconductivity, topological order, and quantum spin liquids (QSLs). A QSL is a quantum phase (QP) related to a quantum-entangled fluid-like state of matter. Previous experiments on QSL candidate materials are usually interpreted in terms of a single QP, although theories indicate that many distinct QPs are closely competing in typical frustrated spin models. Here we report on combined temperature- dependent muon spin relaxation and specific heat measurements for the triangular-lattice QSL candidate material 1T-TaS2 that provide evidence for competing QPs. The measured properties are assigned to arrays of individual QSL layers within the layered charge density wave structure of 1T-TaS2 and their characteristic parameters can be interpreted as those of distinct Z2 QSL phases. The present results reveal that a QSL description can extend beyond the lowest temperatures, offering an additional perspective in the search for such materials. npj Quantum Materials (2021) 6:69 ; https://doi.org/10.1038/s41535-021-00367-w INTRODUCTION QSL, both from experimental and theoretical perspectives13,18–21, The idea of destabilizing magnetic order by quantum fluctuating but some alternative scenarios have also been proposed, such as a 1234567890():,; resonating valence bonds (RVB) originated with Anderson in Peierls mechanism or the formation of domain wall networks, 22–24 19731. Systems with frustrated interactions are particularly among others . -
Polaron Formation in Cuprates
Polaron formation in cuprates Olle Gunnarsson 1. Polaronic behavior in undoped cuprates. a. Is the electron-phonon interaction strong enough? b. Can we describe the photoemission line shape? 2. Does the Coulomb interaction enhance or suppress the electron-phonon interaction? Large difference between electrons and phonons. Cooperation: Oliver Rosch,¨ Giorgio Sangiovanni, Erik Koch, Claudio Castellani and Massimo Capone. Max-Planck Institut, Stuttgart, Germany 1 Important effects of electron-phonon coupling • Photoemission: Kink in nodal direction. • Photoemission: Polaron formation in undoped cuprates. • Strong softening, broadening of half-breathing and apical phonons. • Scanning tunneling microscopy. Isotope effect. MPI-FKF Stuttgart 2 Models Half- Coulomb interaction important. breathing. Here use Hubbard or t-J models. Breathing and apical phonons: Coupling to level energies >> Apical. coupling to hopping integrals. ⇒ g(k, q) ≈ g(q). Rosch¨ and Gunnarsson, PRL 92, 146403 (2004). MPI-FKF Stuttgart 3 Photoemission. Polarons H = ε0c†c + gc†c(b + b†) + ωphb†b. Weak coupling Strong coupling 2 ω 2 ω 2 1.8 (g/ ph) =0.5 (g/ ph) =4.0 1.6 1.4 1.2 ph ω ) 1 ω A( 0.8 0.6 Z 0.4 0.2 0 -8 -6 -4 -2 0 2 4 6-6 -4 -2 0 2 4 ω ω ω ω / ph / ph Strong coupling: Exponentially small quasi-particle weight (here criterion for polarons). Broad, approximately Gaussian side band of phonon satellites. MPI-FKF Stuttgart 4 Polaronic behavior Undoped CaCuO2Cl2. K.M. Shen et al., PRL 93, 267002 (2004). Spectrum very broad (insulator: no electron-hole pair exc.) Shape Gaussian, not like a quasi-particle. -
Hydrodynamics of the Dark Superfluid: II. Photon-Phonon Analogy Marco Fedi
Hydrodynamics of the dark superfluid: II. photon-phonon analogy Marco Fedi To cite this version: Marco Fedi. Hydrodynamics of the dark superfluid: II. photon-phonon analogy. 2017. hal- 01532718v2 HAL Id: hal-01532718 https://hal.archives-ouvertes.fr/hal-01532718v2 Preprint submitted on 28 Jun 2017 (v2), last revised 19 Jul 2017 (v3) HAL is a multi-disciplinary open access L’archive ouverte pluridisciplinaire HAL, est archive for the deposit and dissemination of sci- destinée au dépôt et à la diffusion de documents entific research documents, whether they are pub- scientifiques de niveau recherche, publiés ou non, lished or not. The documents may come from émanant des établissements d’enseignement et de teaching and research institutions in France or recherche français ou étrangers, des laboratoires abroad, or from public or private research centers. publics ou privés. Distributed under a Creative Commons Attribution| 4.0 International License manuscript No. (will be inserted by the editor) Hydrodynamics of the dark superfluid: II. photon-phonon analogy. Marco Fedi Received: date / Accepted: date Abstract In “Hydrodynamic of the dark superfluid: I. gen- have already discussed the possibility that quantum vacu- esis of fundamental particles” we have presented dark en- um be a hydrodynamic manifestation of the dark superflu- ergy as an ubiquitous superfluid which fills the universe. id (DS), [1] which may correspond to mainly dark energy Here we analyze light propagation through this “dark su- with superfluid properties, as a cosmic Bose-Einstein con- perfluid” (which also dark matter would be a hydrodynamic densate [2–8,14]. Dark energy would confer on space the manifestation of) by considering a photon-phonon analogy, features of a superfluid quantum space. -
Multicritical Nambu-Goldstone Modes and Nonrelativistic Naturalness
1 Multicritical Nambu-Goldstone Modes and Nonrelativistic Naturalness Petr Hoˇrava Berkeley Center for Theoretical Physics Bay Area Particle Theory Seminar SFSU, San Francisco, CA October 10, 2014 work with Tom Griffin, Kevin Grosvenor, Ziqi Yan 2 Puzzles of Naturalness Some of the most fascinating open problems in modern physics are all problems of naturalness: • The cosmological constant problem • The Higgs mass hierarchy problem • The linear resistivity of strange metals, the regime above Tc in high-Tc superconductors [Bednorz&M¨uller'86; Polchinski '92] In addition, the first two •s { together with the recent experimental facts { suggest that we may live in a strangely simple Universe ::: Naturalness is again in the forefront (as are its possible alternatives: landscape? :::?) If we are to save naturalness, we need new surprises! 3 What is Naturalness? Technical Naturalness: 't Hooft (1979) \The concept of causality requires that macroscopic phenomena follow from microscopic equations." \The following dogma should be followed: At any energy scale µ, a physical parameter or a set of physical parameters αi(µ) is allowed to be very small only if the replacement αi(µ) = 0 would increase the symmetry of the system." Example: Massive λφ4 in 3 + 1 dimensions. p λ ∼ "; m2 ∼ µ2"; µ ∼ m= λ. Symmetry: The constant shift φ ! φ + a. \Pursuing naturalness beyond 1000 GeV will require theories that are immensely complex compared with some of the grand unified schemes." 4 Gravity without Relativity (a.k.a. gravity with anisotropic scaling, or Hoˇrava-Lifshitz gravity) Field theories with anisotropic scaling: xi ! λxi; t ! λzt: z: dynamical critical exponent { characteristic of RG fixed point. -
Development of Phonon-Mediated Cryogenic
DEVELOPMENT OF PHONON-MEDIATED CRYOGENIC PARTICLE DETECTORS WITH ELECTRON AND NUCLEAR RECOIL DISCRIMINATION a dissertation submitted to the department of physics and the committee on graduate studies of stanford university in partial fulfillment of the requirements for the degree of doctor of philosophy Sae Woo Nam December, 1998 c Copyright 1999 by Sae Woo Nam All Rights Reserved ii I certify that I have read this dissertation and that in my opinion it is fully adequate, in scope and in quality, as a dissertation for the degree of Doctor of Philosophy. Blas Cabrera (Principal Advisor) I certify that I have read this dissertation and that in my opinion it is fully adequate, in scope and in quality, as a dissertation for the degree of Doctor of Philosophy. Douglas Osheroff I certify that I have read this dissertation and that in my opinion it is fully adequate, in scope and in quality, as a dissertation for the degree of Doctor of Philosophy. Roger Romani Approved for the University Committee on Graduate Studies: iii Abstract Observations have shown that galaxies, including our own, are surrounded by halos of "dark matter". One possibility is that this may be an undiscovered form of matter, weakly interacting massive particls (WIMPs). This thesis describes the development of silicon based cryogenic particle detectors designed to directly detect interactions with these WIMPs. These detectors are part of a new class of detectors which are able to reject background events by simultane- ously measuring energy deposited into phonons versus electron hole pairs. By using the phonon sensors with the ionization sensors to compare the partitioning of energy between phonons and ionizations we can discriminate betweeen electron recoil events (background radiation) and nuclear recoil events (dark matter events).