Gas Dynamics and Kinetics in the Cometary Coma: Theory and Observations
Total Page:16
File Type:pdf, Size:1020Kb
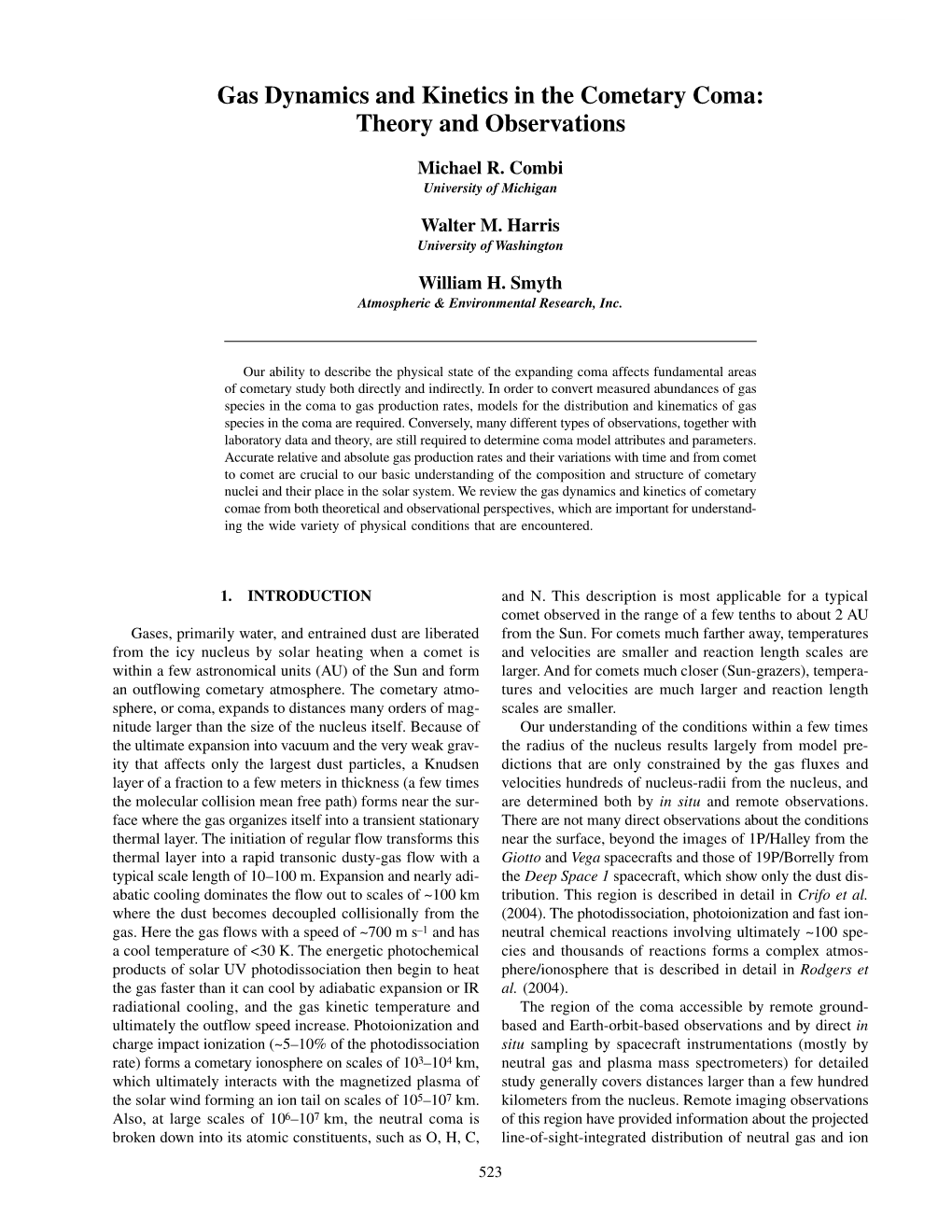
Load more
Recommended publications
-
Astronomy Unusually High Magnetic Fields in the Coma of 67P/Churyumov-Gerasimenko During Its High-Activity Phase C
Astronomy Unusually high magnetic fields in the coma of 67P/Churyumov-Gerasimenko during its high-activity phase C. Goetz, B. Tsurutani, Pierre Henri, M. Volwerk, E. Béhar, N. Edberg, A. Eriksson, R. Goldstein, P. Mokashi, H. Nilsson, et al. To cite this version: C. Goetz, B. Tsurutani, Pierre Henri, M. Volwerk, E. Béhar, et al.. Astronomy Unusually high mag- netic fields in the coma of 67P/Churyumov-Gerasimenko during its high-activity phase. Astronomy and Astrophysics - A&A, EDP Sciences, 2019, 630, pp.A38. 10.1051/0004-6361/201833544. hal- 02401155 HAL Id: hal-02401155 https://hal.archives-ouvertes.fr/hal-02401155 Submitted on 9 Dec 2019 HAL is a multi-disciplinary open access L’archive ouverte pluridisciplinaire HAL, est archive for the deposit and dissemination of sci- destinée au dépôt et à la diffusion de documents entific research documents, whether they are pub- scientifiques de niveau recherche, publiés ou non, lished or not. The documents may come from émanant des établissements d’enseignement et de teaching and research institutions in France or recherche français ou étrangers, des laboratoires abroad, or from public or private research centers. publics ou privés. A&A 630, A38 (2019) Astronomy https://doi.org/10.1051/0004-6361/201833544 & © ESO 2019 Astrophysics Rosetta mission full comet phase results Special issue Unusually high magnetic fields in the coma of 67P/Churyumov-Gerasimenko during its high-activity phase C. Goetz1, B. T. Tsurutani2, P. Henri3, M. Volwerk4, E. Behar5, N. J. T. Edberg6, A. Eriksson6, R. Goldstein7, P. Mokashi7, H. Nilsson4, I. Richter1, A. Wellbrock8, and K. -
Schlieren Imaging of Loud Sounds and Weak Shock Waves in Air Near the Limit of Visibility
Shock Waves DOI 10.1007/s00193-009-0226-6 ORIGINAL ARTICLE Schlieren imaging of loud sounds and weak shock waves in air near the limit of visibility Michael John Hargather · Gary S. Settles · Matthew J. Madalis Received: 27 April 2009 / Accepted: 4 August 2009 © Springer-Verlag 2009 Abstract A large schlieren system with exceptional sensi- Keywords Shock waves · Nonlinear acoustics · Sound · tivity and a high-speed digital camera are used to visualize Noise · Schlieren · High-speed imaging loud sounds and a variety of common phenomena that pro- duce weak shock waves in the atmosphere. Frame rates var- PACS 42.79.Mt · 43.25.+y ied from 10,000 to 30,000frames/s with microsecond frame exposures. Sound waves become visible to this instrumenta- tion at frequencies above 10kHz and sound pressure levels 1 Introduction in the 110dB (6.3Pa) range and above. The density gradient produced by a weak shock wave is examined and found to Visualizing sound-wave motion in air has been a dream of sci- depend upon the profile and thickness of the shock as well as entists and engineers for at least a century and a half, although the density difference across it. Schlieren visualizations of sound waves and weak shock waves were initially confused. weak shock waves from common phenomena include loud August Toepler’s (1836–1912) epitaph, “He was the first to trumpet notes, various impact phenomena that compress a see sound,” actually referred to his schlieren imaging of weak bubble of air, bursting a toy balloon, popping a champagne shock waves from electric sparks [1]. -
Early Observations of the Interstellar Comet 2I/Borisov
geosciences Article Early Observations of the Interstellar Comet 2I/Borisov Chien-Hsiu Lee NSF’s National Optical-Infrared Astronomy Research Laboratory, Tucson, AZ 85719, USA; [email protected]; Tel.: +1-520-318-8368 Received: 26 November 2019; Accepted: 11 December 2019; Published: 17 December 2019 Abstract: 2I/Borisov is the second ever interstellar object (ISO). It is very different from the first ISO ’Oumuamua by showing cometary activities, and hence provides a unique opportunity to study comets that are formed around other stars. Here we present early imaging and spectroscopic follow-ups to study its properties, which reveal an (up to) 5.9 km comet with an extended coma and a short tail. Our spectroscopic data do not reveal any emission lines between 4000–9000 Angstrom; nevertheless, we are able to put an upper limit on the flux of the C2 emission line, suggesting modest cometary activities at early epochs. These properties are similar to comets in the solar system, and suggest that 2I/Borisov—while from another star—is not too different from its solar siblings. Keywords: comets: general; comets: individual (2I/Borisov); solar system: formation 1. Introduction 2I/Borisov was first seen by Gennady Borisov on 30 August 2019. As more observations were conducted in the next few days, there was growing evidence that this might be an interstellar object (ISO), especially its large orbital eccentricity. However, the first astrometric measurements do not have enough timespan and are not of same quality, hence the high eccentricity is yet to be confirmed. This had all changed by 11 September; where more than 100 astrometric measurements over 12 days, Ref [1] pinned down the orbit elements of 2I/Borisov, with an eccentricity of 3.15 ± 0.13, hence confirming the interstellar nature. -
Gas Kinetics in Galactic Disk. Why We Do Not Need a Dark Matter to Explain Rotation Curves of Spirals? Anton Lipovka
Gas kinetics in galactic disk. Why we do not need a dark matter to explain rotation curves of spirals? Anton Lipovka To cite this version: Anton Lipovka. Gas kinetics in galactic disk. Why we do not need a dark matter to explain rotation curves of spirals?. 2018. hal-01832309v3 HAL Id: hal-01832309 https://hal.archives-ouvertes.fr/hal-01832309v3 Preprint submitted on 24 Jul 2020 HAL is a multi-disciplinary open access L’archive ouverte pluridisciplinaire HAL, est archive for the deposit and dissemination of sci- destinée au dépôt et à la diffusion de documents entific research documents, whether they are pub- scientifiques de niveau recherche, publiés ou non, lished or not. The documents may come from émanant des établissements d’enseignement et de teaching and research institutions in France or recherche français ou étrangers, des laboratoires abroad, or from public or private research centers. publics ou privés. Gas kinetics in galactic disk. Why we do not need a dark matter to explain rotation curves of spirals? Lipovka A.A. Department of Research for Physics, Sonora University, 83000, Hermosillo, Sonora, México May 27, 2020 Abstract In present paper, we analyze how the physical properties of gas affect the Rotation Curve (RC) of a spiral galaxy. It is shown, that the observed non-Keplerian RCs measured for outer part of disks, and the observed radial gas distribution are closely related by the diffusion equation, which clearly indicates that no additional mass (Dark Matter) is need. It is stressed, that while the inner part of the RC is subject of the Kepler law, for the correct description of the outer part of the RC, the collisional property of the gas should also be taken into account. -
Interstellar Comet 2I/Borisov Exhibits a Structure Similar to Native Solar System Comets⋆
Mon. Not. R. Astron. Soc. 000, 1–5 (201X) Printed 4 April 2020 (MN LATEX style file v2.2) Interstellar Comet 2I/Borisov exhibits a structure similar to native Solar System comets⋆ F. Manzini1†, V. Oldani1, P. Ochner2,3, and L.R.Bedin2 1Stazione Astronomica di Sozzago, Cascina Guascona, I-28060 Sozzago (Novara), Italy 2INAF-Osservatorio Astronomico di Padova, Vicolo dell’Osservatorio 5, I-35122 Padova, Italy 3Department of Physics and Astronomy-University of Padova, Via F. Marzolo 8, I-35131 Padova, Italy Letter: Accepted 2020 April 1. Received 2020 April 1; in original form 2019 November 22. ABSTRACT We processed images taken with the Hubble Space Telescope (HST) to investigate any morphological features in the inner coma suggestive of a peculiar activity on the nucleus of the interstellar comet 2I/Borisov. The coma shows an evident elongation, in the position angle (PA) ∼0◦-180◦ direction, which appears related to the presence of a jet originating from a single active source on the nucleus. A counterpart of this jet directed towards PA ∼10◦ was detected through analysis of the changes of the inner coma morphology on HST images taken in different dates and processed with different filters. These findings indicate that the nucleus is probably rotating with a spin axis projected near the plane of the sky and oriented at PA ∼100◦-280◦, and that the active source is lying in a near-equatorial position. Subsequent observations of HST allowed us to determine the direction of the spin axis at RA = 17h20m ±15◦ and Dec = −35◦ ±10◦. Photometry of the nucleus on HST images of 12 October 2019 only span ∼7 hours, insufficient to reveal a rotational period. -
Closing the Gap Between Ground Based and In-Situ Observations of Cometary Dust Activity: Investigating Comet 67P to Gain a Deeper Understanding of Other Comets
Closing the gap between ground based and in-situ observations of cometary dust activity: Investigating comet 67P to gain a deeper understanding of other comets. Raphael Marschall & Oleksandra Ivanova et al. Abstract When cometary dust particles are ejected from the surface they are accelerated because of the surrounding gas flow from sublimating ices. As these particles travel millions of kilometres from their origin into the solar system they produce the magnificent tails commonly associated with comets. During their long journey the dust particles transition through different regimes of changing dominant forces such as gas drag, cometary gravity, solar radiation pressure, and solar gravity. The transition and link between the different regimes is to this day poorly understood. There are two main reasons for this. Firstly, the problem covers a vast range in spatial and temporal scales that need to be matched taking into account multiple transitions of the force regime. Furthermore, observational data covering these large spatial and temporal scales for at least one comet and thus characterising it in great detail has been lacking until recently. For comet 67P/Churyumov-Gerasimenko (hereafter 67P) a small number of large scale struc- tures in the outer dust coma and tail have been found from ground based observations. Con- versely ESA's Rosetta mission has shown many small scale structures defining the innermost coma close to the nucleus surface. This disconnect between the observations on these different scales has yet to be understood and explained. Solving this problem thus requires an interdisciplinary approach. This ISSI team shall bring together experts of the recent observational data from ground and in-situ of comet 67P as well as theorists that are able to model the dynamical processes over these different scales. -
Project RAND Publication: RA-15022
, , ,-.. ,'. --. - ~.-~~ UNCLASSIFIED (rA) AERODYNAMICS, GAS DYNAMICS AND HEAT TRANSFER PROBLEMS OF A SATELLITE ROCKET RA-15022 February 1, 1947 UNCLASSI FI ED DOUGLAS AIRCRAFT COMPANY, INC. == DECLASSIFIED lAW E012958 BY EO Review Team DATE &' - ) r; --- :J-cJl r} This document contains information affecting the national defense of the United States within the meaning of the Espionage Act, SO U.S.C., 31 and 32, as amended. Its transmission or the revelation of its contents in any manner to an unauthorized person is prohibited by law. SECRET TABLE OF CONTENTS LIST OF FIGURES ...... , ......................................................... v SUMMARy .........................................................................vii LIST OF SyMBOLS ............................................................... ix I. AERODYNAMICS .............................................................. I A. Drag ....................................................................... l 1. The Drag Forces Exerted on a Supersonic Rocket ......................... 1 2. Approximations Used in the Drag Analysis ............................... 4 3. Methods Employed in Evaluating the Drag ................................ 6 4. The Optimum Shape for a Satellite Rocket ......... , ..................... 8 5. Rarefied Gas Dynamics (Superaerodynamics) ............................. 20 B. Aerodynamics of Stability and Control. .. , ..............•.... : ............. 20 L Control Manent ..................................... '............ , ...... 21 2. Body Moment .......................................................... -
FIRST COMETARY OBSERVATIONS with ALMA: C/2012 F6 (LEMMON) and C/2012 S1 (ISON) Martin Cordiner1,2, Stefanie Milam1, Michael Mumm
45th Lunar and Planetary Science Conference (2014) 2609.pdf FIRST COMETARY OBSERVATIONS WITH ALMA: C/2012 F6 (LEMMON) AND C/2012 S1 (ISON) Martin Cordiner1,2, Stefanie Milam1, Michael Mumma1, Steven Charnley1, Anthony Remijan3, Geronimo Vil- lanueva1,2, Lucas Paganini1,2, Jeremie Boissier4, Dominique Bockelee-Morvan5, Nicolas Biver5, Dariusz Lis6, Yi- Jehng Kuan7, Jacques Crovisier5, Iain Coulson9, Dante Minniti10 1Goddard Center for Astrobiology, NASA Goddard Space Flight Center, 8800 Greenbelt Road, Greenbelt, MD 20770, USA; email: [email protected]. 2The Catholic University of America, 620 Michigan Ave. NE, Washington, DC 20064, USA. 3NRAO, Charlottesville, VA 22903, USA. 4IRAM, 300 Rue de la Piscine, 38406 Saint Martin d’Heres, France. 5Observatoire de Paris, Meudon, France. 6Caltech, Pasadena, CA 91125, USA. 7National Taiwan Normal University, Taipei, Taiwan. 9Joint As- tronomy Centre, Hilo, HI 96720, USA. 10Pontifica Universidad Catolica de Chile, Santigo, Chile. Introduction: Cometary ices are believed to have The observed visibilities were flagged, calibrated aggregated around the time the Solar System formed and imaged using standard routines in the NRAO (c. 4.5 Gyr ago), and have remained in a frozen, rela- CASA software (version 4.1.0). Image deconvolution tively quiescent state ever since. Ground-based studies was performed using the Hogbom algorithm with natu- of the compositions of cometary comae provide indi- ral weighting and a threshold flux level of twice the rect information on the compositions of the nuclear RMS noise. ices, and thus provide insight into the physical and chemical conditions of the early Solar Nebula. To real- Results: The data cubes show spectrally and spa- ize the full potential of gas-phase coma observations as tially-resolved structures in HCN, CH3OH and H2CO probes of solar system evolution therefore requires a emission in both comets, consistent with anisotropic complete understanding of the gas-release mecha- outgassing from their nuclei. -
Research Paper in Nature
Draft version November 1, 2017 Typeset using LATEX twocolumn style in AASTeX61 DISCOVERY AND CHARACTERIZATION OF THE FIRST KNOWN INTERSTELLAR OBJECT Karen J. Meech,1 Robert Weryk,1 Marco Micheli,2, 3 Jan T. Kleyna,1 Olivier Hainaut,4 Robert Jedicke,1 Richard J. Wainscoat,1 Kenneth C. Chambers,1 Jacqueline V. Keane,1 Andreea Petric,1 Larry Denneau,1 Eugene Magnier,1 Mark E. Huber,1 Heather Flewelling,1 Chris Waters,1 Eva Schunova-Lilly,1 and Serge Chastel1 1Institute for Astronomy, 2680 Woodlawn Drive, Honolulu, HI 96822, USA 2ESA SSA-NEO Coordination Centre, Largo Galileo Galilei, 1, 00044 Frascati (RM), Italy 3INAF - Osservatorio Astronomico di Roma, Via Frascati, 33, 00040 Monte Porzio Catone (RM), Italy 4European Southern Observatory, Karl-Schwarzschild-Strasse 2, D-85748 Garching bei M¨unchen,Germany (Received November 1, 2017; Revised TBD, 2017; Accepted TBD, 2017) Submitted to Nature ABSTRACT Nature Letters have no abstracts. Keywords: asteroids: individual (A/2017 U1) | comets: interstellar Corresponding author: Karen J. Meech [email protected] 2 Meech et al. 1. SUMMARY 22 confirmed that this object is unique, with the highest 29 Until very recently, all ∼750 000 known aster- known hyperbolic eccentricity of 1:188 ± 0:016 . Data oids and comets originated in our own solar sys- obtained by our team and other researchers between Oc- tem. These small bodies are made of primor- tober 14{29 refined its orbital eccentricity to a level of dial material, and knowledge of their composi- precision that confirms the hyperbolic nature at ∼ 300σ. tion, size distribution, and orbital dynamics is Designated as A/2017 U1, this object is clearly from essential for understanding the origin and evo- outside our solar system (Figure2). -
The Castalia Mission to Main Belt Comet 133P/Elst-Pizarro C
The Castalia mission to Main Belt Comet 133P/Elst-Pizarro C. Snodgrass, G.H. Jones, H. Boehnhardt, A. Gibbings, M. Homeister, N. Andre, P. Beck, M.S. Bentley, I. Bertini, N. Bowles, et al. To cite this version: C. Snodgrass, G.H. Jones, H. Boehnhardt, A. Gibbings, M. Homeister, et al.. The Castalia mission to Main Belt Comet 133P/Elst-Pizarro. Advances in Space Research, Elsevier, 2018, 62 (8), pp.1947- 1976. 10.1016/j.asr.2017.09.011. hal-02350051 HAL Id: hal-02350051 https://hal.archives-ouvertes.fr/hal-02350051 Submitted on 28 Aug 2020 HAL is a multi-disciplinary open access L’archive ouverte pluridisciplinaire HAL, est archive for the deposit and dissemination of sci- destinée au dépôt et à la diffusion de documents entific research documents, whether they are pub- scientifiques de niveau recherche, publiés ou non, lished or not. The documents may come from émanant des établissements d’enseignement et de teaching and research institutions in France or recherche français ou étrangers, des laboratoires abroad, or from public or private research centers. publics ou privés. Distributed under a Creative Commons Attribution| 4.0 International License Available online at www.sciencedirect.com ScienceDirect Advances in Space Research 62 (2018) 1947–1976 www.elsevier.com/locate/asr The Castalia mission to Main Belt Comet 133P/Elst-Pizarro C. Snodgrass a,⇑, G.H. Jones b, H. Boehnhardt c, A. Gibbings d, M. Homeister d, N. Andre e, P. Beck f, M.S. Bentley g, I. Bertini h, N. Bowles i, M.T. Capria j, C. Carr k, M. -
The Distribution of Gases in the Coma of Comet 67P/Churyumov-Gerasimenko from Rosetta Measurements
46th Lunar and Planetary Science Conference (2015) 1714.pdf THE DISTRIBUTION OF GASES IN THE COMA OF COMET 67P/CHURYUMOV-GERASIMENKO FROM ROSETTA MEASUREMENTS. M.R. Combi1, N. Fougere1, V. Tenishev1, A. Bieler1, K. Altwegg2, J.J. Bérthelier3 , J. De Keyser4, B. Fiethe5 , S. A. Fuselier6, T.I. Gombosi1, K.C. Hansen1, M. Hässig6,, Z. Huang1, X. Jia1, M. Rubin2, G. Toth1, Y. Shou1, C.-Y. Tzou2, and the Rosetta ROSINA Science Team. 1Department of Atmos- pheric, Oceanic and Space Science, Unibersity of Michigan, Ann Arbor, MI, [email protected]. 2Physikalisches Institut, University of Bern, Sidlerstr. 5, CH-3012 Bern, Switzerland, Belgian Institute for Space Aeronomy, 3LATMOS/IPSL-CNRS-UPMC-UVSQ, 4 Avenue de Neptune F-94100 SAINT-MAUR, France, 4BIRA-IASB, 5 Ringlaan 3, B-1180 Brussels, Belgium, Institute of Computer and Network Engineering (IDA), TU Braunschweig, 6 Hans-Sommer-Straße 66, D-38106 Braunschweig, Germany, Southwest Research Institute, 6220 Culebra Rd., San Antonio, TX 78238, USA.. Introduction: Since its orbit insertion around • The evolution of the system is simulated by comet 67P/Churyumov-Gerasimenko (CG), the Roset- tracing the model particles ta spacecraft has revealed invaluable information re- • Realistic modeling of collisions in rarefied gas garding the cometary coma environment. The extended • Photochemical reactions for production of the period of observation enables a relatively extensive minor species spatial and temporal coverage of comet CG’s coma, • Two phase simulation: gas and dust in a single which showed distinct distributions for different spe- model run cies and activity on the surface in response to solar • Adaptive mesh with cut-cells illumination as the nucleus rotates. -
Gas Phase Reaction Kinetics of Neutral Oxygen Species
National Bureau ot Standards Library, £01 Admin. Bldg. FEB 3 1969 A11102 mbQQb NSRDS-NBS 20 All 1021 46006 /NSflOS-MI? N6S-.UB-CW NBS PUBLICATIONS Gas Phase Reaction Kinetics Of Neutral Oxygen Species U.S. DEPARTMENT OF COMMERCE NATIONAL BUREAU OF STANDARDS National Standard Reference Data Series National Bureau of Standards National Standard Reference Data System, Plan of Operation, NSRDS-NBS 1 — 15 cents* Thermal Properties of Aqueous Uni-univalent Electrolytes, NSRDS-NBS 2 — 45 cents* Selected Tables of Atomic Spectra, Atomic Energy Levels and Multiplet Tables — Si II, Si III, Si IV, NSRDS-NBS 3, Section 1 — 35 cents* Selected Tables of Atomic Spectra, Atomic Energy Levels and Multiplet Tables — Si I, NSRDS-NBS 3, Section 2 — 20 cents* Atomic Transition Probabilities, Volume I, Hydrogen Through Neon, NSRDS-NBS 4 — $2.50* The Band Spectrum of Carbon Monoxide, NSRDS-NBS 5 — 70 cents* Tables of Molecular Vibrational Frequencies, Part 1, NSRDS-NBS 6 — 40 cents* High Temperature Properties and Decomposition of Inorganic Salts, Part 1, Sulfates, NSRDS-NBS 7-35 cents* Thermal Conductivity of Selected Materials, NSRDS-NBS 8 — 11.00* Tables of Bimolecular Gas Reactions, NSRDS-NBS 9 — $2.00* Selected Values of Electric Dipole Moments for Molecules in the Gas Phase, NSRDS- NBS 10-40 cents* Tables of Molecular Vibrational Frequencies, Part 2, NSRDS-NBS 11 — 30 cents* Tables for the Rigid Asymmetric Rotor: Transformation Coefficient for Symmetric to Asymmetric Bases and Expectation Values of P§, P|, and Pf, NSRDS-NBS 12 — 60 cents* Hydrogenation