Hydrous Silicates and Water on Venus
Total Page:16
File Type:pdf, Size:1020Kb
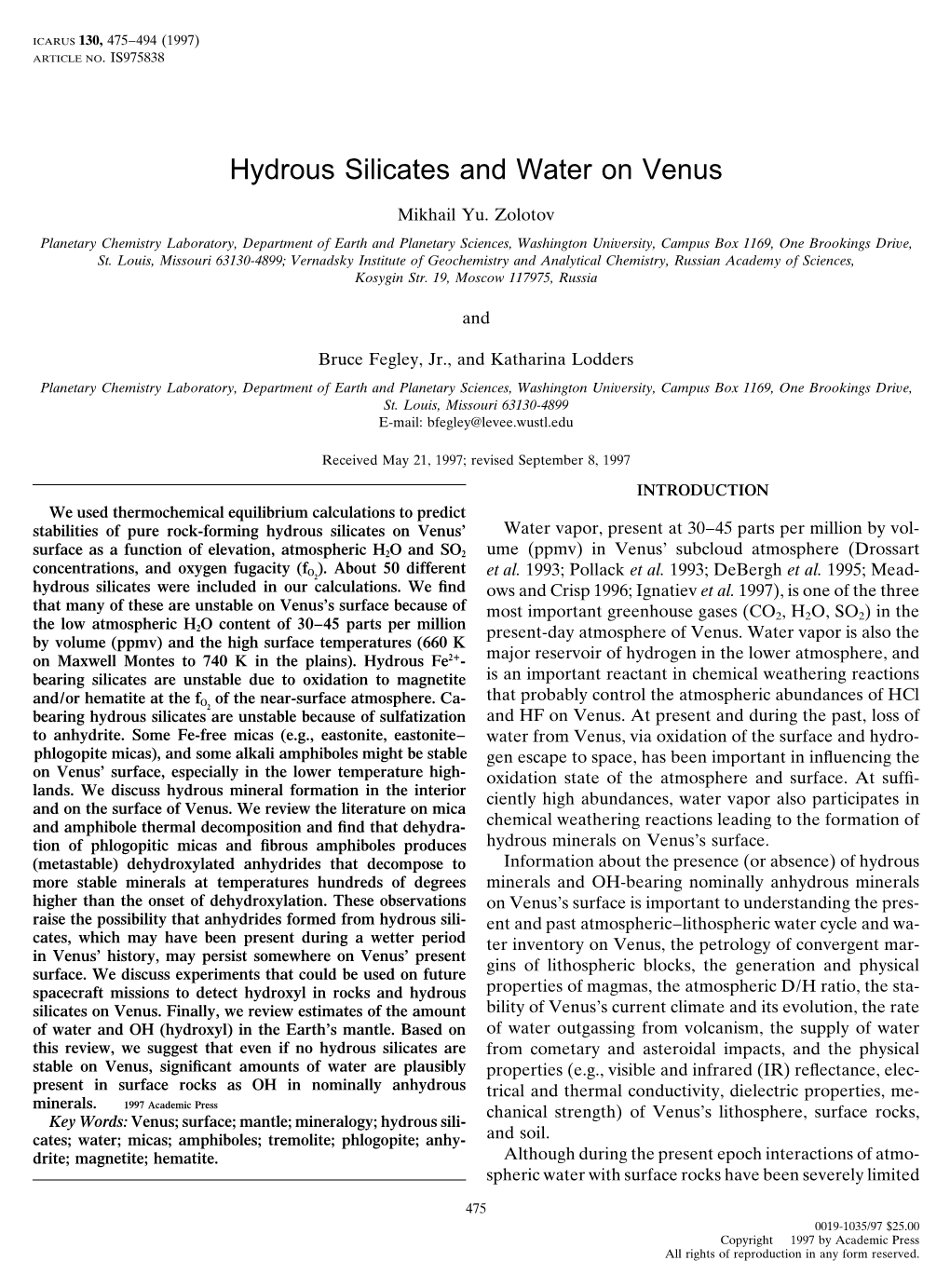
Load more
Recommended publications
-
Electrons, Life and the Evolution of Earth's Chemical Cycles
Electrons, Life and the Evolution of Earth’s Chemical Cycles OCN 623 – Chemical Oceanography 23 April 2013 Adaption of Brian Glazer lecture (based on Falkowski and Godfrey (2008) Phil. Trans. R. Soc. B, 363: 2705-2716) © 2013 F.J. Sansone Outline Earth’s geological, geochemical and biological co- evolution since formation - Early Earth - Origins of life - The great oxidation event - Linkage between global O and N cycles - Alternate explanations for the great oxidation event - The rise of oxygen and the evolution of life - The Phanerozoic How Does the Earth Work as a Biosphere? All organisms derive energy for growth and maintenance by moving electrons from a substrate to a product All substrates and products must ultimately be recycled All metabolic processes on Earth are prokaryotic and were developed in the Archean and/or Proterozoic Eons Earth’s Geological, Geochemical and Biological Co-evolution Since Formation Earth, ~3.5 Ga Ago • Earth cools to <100˚C • Shallow sea environment – Land covered by low egg- shaped hills and pillow lavas – Silt layers – Scattered volcanic islands and evaporite lagoons • Tides higher – Moon closer to Earth, days shorter • Atmosphere –CO2-rich, no O2 – UV-drenched landscape Faint Sun Paradox High atmospheric CO2 and CH4 important in early Earth -- balanced weak Sun to maintain temp Recycling of Atmospheric CO2 Liquid water on early Earth allowed a hydrologic cycle, carbonate and silicate mineral weathering to develop: 2+ - CaCO3 + CO2 + H2O = Ca + 2HCO3 2+ - CaSiO3 +2CO2 +3H2O = Ca + 2HCO3 + H4SIO4 Uptake -
COURT of CLAIMS of THE
REPORTS OF Cases Argued and Determined IN THE COURT of CLAIMS OF THE STATE OF ILLINOIS VOLUME 39 Containing cases in which opinions were filed and orders of dismissal entered, without opinion for: Fiscal Year 1987 - July 1, 1986-June 30, 1987 SPRINGFIELD, ILLINOIS 1988 (Printed by authority of the State of Illinois) (65655--300-7/88) PREFACE The opinions of the Court of Claims reported herein are published by authority of the provisions of Section 18 of the Court of Claims Act, Ill. Rev. Stat. 1987, ch. 37, par. 439.1 et seq. The Court of Claims has exclusive jurisdiction to hear and determine the following matters: (a) all claims against the State of Illinois founded upon any law of the State, or upon an regulation thereunder by an executive or administrative ofgcer or agency, other than claims arising under the Workers’ Compensation Act or the Workers’ Occupational Diseases Act, or claims for certain expenses in civil litigation, (b) all claims against the State founded upon any contract entered into with the State, (c) all claims against the State for time unjustly served in prisons of this State where the persons imprisoned shall receive a pardon from the Governor stating that such pardon is issued on the grounds of innocence of the crime for which they were imprisoned, (d) all claims against the State in cases sounding in tort, (e) all claims for recoupment made by the State against any Claimant, (f) certain claims to compel replacement of a lost or destroyed State warrant, (g) certain claims based on torts by escaped inmates of State institutions, (h) certain representation and indemnification cases, (i) all claims pursuant to the Law Enforcement Officers, Civil Defense Workers, Civil Air Patrol Members, Paramedics and Firemen Compensation Act, (j) all claims pursuant to the Illinois National Guardsman’s and Naval Militiaman’s Compensation Act, and (k) all claims pursuant to the Crime Victims Compensation Act. -
Is Extraterrestrial Organic Matter Relevant to the Origin of Life on Earth?
IS EXTRATERRESTRIAL ORGANIC MATTER RELEVANT TO THE ORIGIN OF LIFE ON EARTH? D. C. B. WHITTET Department of Physics, Applied Physics and Astronomy, Rensselaer Polytechnic Institute, Troy, NY 12180, U.S.A. (Received 19 August 1996) Abstract. I review the relative importance of internal and external sources of prebiotic molecules on Earth at the time of life's origin 3.7 Gyr ago. The ef®ciency of synthesis in the Earth's atmosphere was critically dependent on its oxidation state. If the early atmosphere was non-reducing and CO2- dominated, external delivery might have been the dominant source. Interplanetary dust grains and micrometeorites currently deliver carbonaceous matter to the Earth's surface at a rate of 3 5 7 10 kg/yr (equivalent to a biomass in 2 Gyr), but this may have been as high as 5 10 kg/yr (a biomass in only 10 Myr) during the epoch of late bombardment. Much of the incoming material is in the form of chemically inactive kerogens and amorphous carbon; but if the Earth once had a dense (10-bar) atmosphere, small comets rich in a variety of prebiotic molecules may have been suf®ciently air-braked to land non-destructively. Lingering uncertainties regarding the impact history of the Earth and the density and composition of its early atmosphere limit our ability to draw ®rm conclusions. 1. Introduction In at least one sense, a connection between the Universe at large and life in our small corner of it is inevitable. The hydrogen, carbon, nitrogen, oxygen, and other elements that make up our bodies and other living things were created billions of years ago in the interiors of stars and, in the case of hydrogen, in the the Big Bang itself (see Trimble, 1997, in this volume for an eloquent review). -
Investigating Mineral Stability Under Venus Conditions: a Focus on the Venus Radar Anomalies Erika Kohler University of Arkansas, Fayetteville
University of Arkansas, Fayetteville ScholarWorks@UARK Theses and Dissertations 5-2016 Investigating Mineral Stability under Venus Conditions: A Focus on the Venus Radar Anomalies Erika Kohler University of Arkansas, Fayetteville Follow this and additional works at: http://scholarworks.uark.edu/etd Part of the Geochemistry Commons, Mineral Physics Commons, and the The unS and the Solar System Commons Recommended Citation Kohler, Erika, "Investigating Mineral Stability under Venus Conditions: A Focus on the Venus Radar Anomalies" (2016). Theses and Dissertations. 1473. http://scholarworks.uark.edu/etd/1473 This Dissertation is brought to you for free and open access by ScholarWorks@UARK. It has been accepted for inclusion in Theses and Dissertations by an authorized administrator of ScholarWorks@UARK. For more information, please contact [email protected], [email protected]. Investigating Mineral Stability under Venus Conditions: A Focus on the Venus Radar Anomalies A dissertation submitted in partial fulfillment of the requirements for the degree of Doctor of Philosophy in Space and Planetary Sciences by Erika Kohler University of Oklahoma Bachelors of Science in Meteorology, 2010 May 2016 University of Arkansas This dissertation is approved for recommendation to the Graduate Council. ____________________________ Dr. Claud H. Sandberg Lacy Dissertation Director Committee Co-Chair ____________________________ ___________________________ Dr. Vincent Chevrier Dr. Larry Roe Committee Co-chair Committee Member ____________________________ ___________________________ Dr. John Dixon Dr. Richard Ulrich Committee Member Committee Member Abstract Radar studies of the surface of Venus have identified regions with high radar reflectivity concentrated in the Venusian highlands: between 2.5 and 4.75 km above a planetary radius of 6051 km, though it varies with latitude. -
Styles of Deformation in Ishtar Terra and Their Implications
JOURNAL OF GEOPHYSICAL RESEARCH, VOL. 97, NO. El0, PAGES 16,085-16,120, OCTOBER 25, 1992 Stylesof Deformationin IshtarTerra and Their Implications Wn.T.TAMM. KAU•A,• DOAN•L. BINDSCHAD•-R,l ROBERT E. GPaM•,2'3 VICKIL. HANSEN,2KARl M. ROBERTS,4AND SUZANNE E. SMREr,AR s IshtarTerra, the highest region on Venus, appears to havecharacteristics of both plume uplifts and convergent belts.Magellan imagery over longitudes 330ø-30øE indicates a great variety of tectonicand volcanic activity, with largevariations within distances of onlya few 100km. Themost prominent terrain types are the volcanic plains of Lakshmiand the mountain belts of Maxwell,Freyja, and Danu. Thebelts appear to havemarked variations in age. Thereare also extensive regions of tesserain boththe upland and outboard plateaus, some rather featureless smoothscarps, flanking basins of complexextensional tectonics, and regions of gravitationalor impactmodifica- tion.Parts of Ishtarare the locations of contemporaryvigorous tectonics and past extensive volcanism. Ishtar appearsto be the consequence of a history of several100 m.y., in whichthere have been marked changes in kinematic patternsand in whichactivity at any stage has been strongly influenced by the past. Ishtar demonstrates three general propertiesof Venus:(1) erosionaldegradation is absent,leading to preservationof patternsresulting from past activity;(2) manysurface features are the responses ofa competentlayer less than 10 km thick to flowsof 100km orbroaderscale; and (3) thesebroader scale flows are controlled mainly by heterogeneities inthe mantle. Ishtar Terra doesnot appear to bethe result of a compressionconveyed by anEarthlike lithosphere. But there is stilldoubt as to whetherIshtar is predominantlythe consequence of a mantleupflow or downflow.Upflow is favoredby the extensivevolcanic plain of Lakshmiand the high geoid: topography ratio; downflow is favoredby the intense deformationof themountain belts and the absence of majorrifts. -
Life in the Outer Solar System Jupiter
Life in the Outer Solar System Jupiter Big R = 11R⊕ Massive M = 300 M⊕ = 2.5 all the rest Thick Atmosphere Mostly H2, He But also more complex molecules Colors, storms Like Miller - Urey Life in Jupiter Atmosphere? Sagan-Salpeter, etc. Sinkers (Plankton) Floaters (Fish) Hunters (Fish) Galileo Results on Jupiter Reached Jupiter Dec. 1995 Sent probe into Jupiter’s atmosphere at 100,000 mile/hour Decelerated at 230 g Lasted for 57 min. Found: Strong winds Turbulence, little lightning Life less likely? Surprise: Little or no H2O May have entered in an unusual place (fewer clouds) Europa (Moon of Jupiter) Surface: Fractured Ice Subsurface Oceans? (Heated from Inside) Close-up of “ice floes” Galileo - Jupiter’s Moons http://www.jpl.nasa.gov/galileo/index.html Europa has a (THIN!) atmosphere UV H H H O=O T hin O O2 H2O ATM Pressure ~ 10–11 Earth More evidence for resurfacing along cracks by “ice geysers” fluid ice or liquid water Organic molecules on Callisto & Ganymede, maybe Europa? Saturn • Big (9.4 R⊕) • Massive (95 M⊕) • Year 29.5 years • Day 0.43 days • Composition similar to Jupiter Titan • Moon of Saturn • Diameter ~0.4 Earth • Atmospheric Pressure = 1.5 × Earth • 85% Nitrogen BUT • Cold (~90 K) • Reducing atmosphere • Haze • Lab for prebiotic chemistry The Cassini-Huygens Mission • Launched 10/13/97 • Arrived Saturn 7/2004 • Cassini studies – Saturn – Moons • Huygens – Dropped onto Titan – Study atmosphere – Surface CASSINI SPACECRAFT Huygens Probe • Released from Cassini • Slowed by heat shield • Parachute deploys • Soft landing • Sample -
Venus' Ishtar Terra: Topographic Analysis of Maxwell, Freyja, Akna
16th VEXAG Meeting 2018 (LPI Contrib. No. 2137) 8046.pdf Venus’ Ishtar Terra: Topographic Analysis of Maxwell, Freyja, Akna and Danu Montes. Sara Rastegar1 and 2 1 Donna M. Jurdy , C ity Colleges of Chicago, Harold Washington College, 30 E. Lake Street, Chicago, IL 60601, 2 [email protected], D epartment of Earth and Planetary Sciences, Northwestern University, 2145 Sheridan Rd, Evanston, IL 60208, [email protected] Introduction: Venus’ mountain chains (Figure 1) Analysis: We attempt to address these questions surround Lakshmi Planum, a 3-4 km highland, making with analysis of Magellan topographic data for up Ishtar Terra. No other mountain belts exist on quantitative comparison of Venus' four mountain Venus. Circling Ishtar Terra, Maxwell Montes, ascends chains: Maxwell, Freyja, Akna and Danu. Patterns in to over 11 km, ranking as the location of highest topography may provide clues to the dynamics forming elevation on the planet. Freyja Montes rises to over 7 these Venusian orogenic belts. From topographic km, higher than Akna Montes at about an elevation of profiles across the ec mountain chain, we then 6 km. Danu Montes, ~1.5 km over Lakshmi Planum, determine an average profile for each mountain belt. alone displays a distinctly arcuate form. Next, we correlate these averages to establish a measure of similarity between the chains and terrestrial Tectonic Enigma: The existence of the four analogs. These correlations allow construction of a venusian mountain chains has been attributed to covariance matrix, which can be diagonalized for localized downwelling - analogous to terrestrial eigenvalues, for Principal Component Analysis (PCA) subduction - in response to the proposed upwelling [4]. -
O Lunar and Planetary Institute Provided by the NASA Astrophysics Data System 1516 Lpscmv LARGE FLOOR-FRACTURED CRATERS: R.W
LPSCXXIV 1515 LARGE FLOOR-FRACTURED CRATERS AND ISOSTATIC CRATER MODIFICATION: IMPLICATIONS FOR LITHOSPHERIC THICKNESS ON VENUS. R.W. Wichman, Dept. of Space Studies, Univ. of North Dakota, Grand Forks, ND 58202-7306 and P.H. Schultz, Dept. of Geological Sciences, Brown Univ., Providence RI 02912 Introduction: Several of the largest craters on Venus, including Mead, Meitner and Isabella, exhibit well-developed floor fracture patterns combining a central set of radial features with a peripheral set of concentric fractures. This pattern strongly resembles the fracture patterns observed in the largest floor- fractured craters on the Moon (eg. Humboldt, Gauss, Petavius). Although most lunar floor-fractured craters apparently reflect crater modification by igneous intrusions and volcanism [I,2,3], we propose that the fractures in these larger craters represent domical flexure events in response to post-impact isostatic uplift. Since the extent of uplift and surface failure in this model depends on both the size of the basin cavity and the local lithospheric thickness, this interpretation also provides a means for constraining lithospheric thicknesses on Venus. Based on the apparent onset diameter of isostatic crater modification, we derive lithospheric thickness estimates for the Moon of -80-100 km, and for Venus of -50-70 km. Large Floor-fractured Craters: As noted in a companion abstract [4], ten craters on Venus show patterns of concentric or polygonal fractures resembling failure patterns observed in lunar floor-fractured craters. These craters are comparable in size (-20-90 km) to most of the lunar examples, and three craters in particular contain well-defined, moat-like structures around a scarp-bounded central floor plate identical to features observed in the most extensively modified craters on the Moon [5]. -
INDEX to VOLUME 26 of the JOURNAL of the ASSOCIATION of LUNAR and PLANETARY OBSERVERS (The Strolling Astronomer) by Michael
INDEX TO VOLUME 26 OF THE JOURNAL OF THE ASSOCIATION OF LUNAR AND PLANETARY OBSERVERS (The Strolling Astronomer) by Michael Mattei PUBLICATION DATA Issue Number 1-2, May, 1976.......Pages 1-40 3-4, August, 1976..... 41-84 5-6, November, 1976... 85-128 7-8, February, 1977... 129-172 9-10, May, 1977........ 173-216 11-12, August, 1977..... 217-260 AUTHOR INDEX Pages Ashbrook, Joseph An Amateur Program: Timing Eclipses of Jovian Satellites 233-235 Benton, Julius L. American and Italian Observations of Saturn in 1974-1975: Some Comparative Notes 166-168 A Simultaneous Observing Program for the Planet Saturn: Some Preliminary Remarks 164-166 Visual and Photographic Observations of Saturn: The 1974-75 Apparition 85-94 The 1975-76 Apparition of Saturn 173-184 The Planet Venus: A Summary of Five Morning Apparitions, 1967 - 1974 150-155 Two Eastern (Evening) Apparitions of the Planet Venus: 1973-74 and 1974-75 100-109 The 1976-77 Eastern (Evening) Apparition of the Planet Venus: Visual and Photographic Investigations 240-251 Budine, Phillip W. Jupiter in 1974-75: Rotation Periods 129-135 Jupiter in 1975-76: Rotation Periods 217-231 Measured Photographic Latitudes on Jupiter in 1975-76 231-233 Observed Latitudes of Jupiter's Belts and Zones in 1974-75 118-119 The A.L.P.O. at Kutztown, Pennsylvania 123-125 Capen, C.F. A Season for Vikings 41-46 Capen, Charles F. and Rhoads, Robert B. Mars 1971 Apparition - Martian Polar Hoods - ALPO Report II 1-8 Sassone-Corsi, Emilio and Sassone- Corsi, Paolo Some Systematic Observations of Saturn During its 1974-75 Apparition 8-12 Delano, Kenneth J. -
NASA GSFC Opportunities for Adult Learning Using the Vantage Point
NASA GSFC OPPORTUNITIES FOR STEM PROFESSIONALS USING THE VANTAGE POINT OF SPACE Blanche W. Meeson§* and Geraldine B. Robbins‡ §NASA Goddard Space Flight Center, Code 610.2/160.00, Greenbelt, MD 20771 ‡Triangle Coalition for Science and Technology Education, NASA Goddard Space Flight Center, Code 160, Greenbelt, MD 20771 ABSTRACT NASA has a variety of learning opportunities for STEM professionals. Three opportunities at GSFC are examined in this chapter: 1) standard summer research and development internship for undergraduates, 2) senior internship for undergraduate and graduate students and 3) a workshop series for informal learning center professionals. We describe these programs, examine their evolution with respect to most effective education practices and their assessment and evaluation, and identify the similarities and differences between them. The internship programs highlight authentic project-based research and development experiences with the senior internship providing a richer, deeper, and more demanding experience that has greater professional value. The workshops for informal learning center professionals on-the-other hand, focus on building knowledge of GSFC’s science and engineering strengths among these professionals, and on building enduring partnerships between individuals (participants and GSFC scientist, engineers and educators) and between organizations (GSFC and the informal learning center). Finally, we examine the characteristics of these programs from a design and management perspective. Through this examination we identify a general structure that provides insight into more effective design and management of similar education programs. INTRODUCTION Learning opportunities have been a key part of NASA’s educational activities since the beginning of the agency. When NASA was established in 1958, its charter directed the agency to provide for the widest practicable and appropriate dissemination of information concerning its activities and the result thereof [1]. -
The Covid Correspondent
The 13 Covid-19 Correspondent Published by The Inchmarlo Social Forum Proprietor: Della Russell Editor: Phil Allen Issue 13: 17 June 2020 Special Edition: Scotland in Lockdown Dolphins have returned to Italy! Meanwhile in Scotland… Scotland in Spring Scotland in Summer Scotland in Autumn Scotland in Lockdown Scottish kids return to school after home-taught history lessons Lockdown Requiem Mr and Mrs Skene’s Cure for Lockdown Blues Sitting, thinking, watching, waiting, (Entertainment Video of the Week) Day of week and month of year, Instructions: Copy & paste the blue text below into your browser’s address Twelve weeks in with more impending, bar. Alternatively place the curser over the blue text and hold down the Brave Scotland's lockdown, Requiem. control key whilst clicking with the mouse. (This could avoid the ads.) https://www.youtube.com/watch?v=KTlMj9EgFDM Do`s and don'ts with `S` all starting Amazing! A new meaning to the phrase “opera glasses” Stay at home, away, apart, Thank you, Alison and Charles Skene. I hope you approve this version. Social distance, pole dependent, `S` word for living, nowhere now. Scottish Banter: • I was out for a walk in Crathes Castle gardens and saw the Coronavirus New friends discovered, neighbours helping, heading towards me. Thanks to the government advice of staying Disciplined folk, bonding, proud, alert, I hid behind a tree and it totally missed me. Thanks Boris. Old friends appear in weekly shopping, • Two people found sunbathing in Scotland today have tested for Remy, Gordon, Jack et. al. hypothermia. • An Englishman, an Irishman and a Scotsman walk into a bar. -
Interview with Harold Zirin
HAROLD ZIRIN (1930-2012) INTERVIEWED BY SHIRLEY K. COHEN February 3, 10 and 17, 1998 Photo taken 1977 ARCHIVES CALIFORNIA INSTITUTE OF TECHNOLOGY Pasadena, California Subject area Astronomy, astrophysics Abstract An interview in three sessions, in February 1998, by Shirley K. Cohen with Harold Zirin, Professor of Astrophysics, emeritus, in the Division of Physics, Math and Astronomy at Caltech. Dr. Zirin received his undergraduate and graduate education at Harvard (AB, 1950; AM, 1951; PhD, 1953). He joined the Caltech faculty in 1964, became Chief Astronomer at the Big Bear Solar Observatory in 1970 and Director in 1980. The interview briefly covers Zirin’s youth and early education in New York City and Bridgeport, Connecticut, and notes his youthful interest in astronomy and success in school. Recalls Harvard astronomers Bart Bok, Harlow Shapley, Armin Deutsch, Donald Menzel. PhD work on stellar opacities under Philip Morse at MIT with Harvard’s approval; leads to first job at RAND Corporation and first move to California, 1952-1953. Denial of security clearance based on father’s membership in Communist party sends him back to Harvard for postdoc position. Move to Colorado to High Altitude Observatory and beginning of solar http://resolver.caltech.edu/CaltechOH:OH_Zirin_H observing; reminiscences of S. Chandrasekhar, G. Munch. Recruitment to Caltech by J. Greenstein, R. Leighton, 1964. Discusses history of solar observing at Mt. Wilson Observatory. Site survey for new Caltech solar observatory; role of astronomer Sue Kiefer; selection of Big Bear Lake site in San Bernardino Mountains (1967). Story of construction and operation of Big Bear Solar Observatory, concluding with its transfer to New Jersey Institute of Technology (1997).