How Are Methane Hydrates Formed, Preserved, and Released? Andrew Lonero
Total Page:16
File Type:pdf, Size:1020Kb
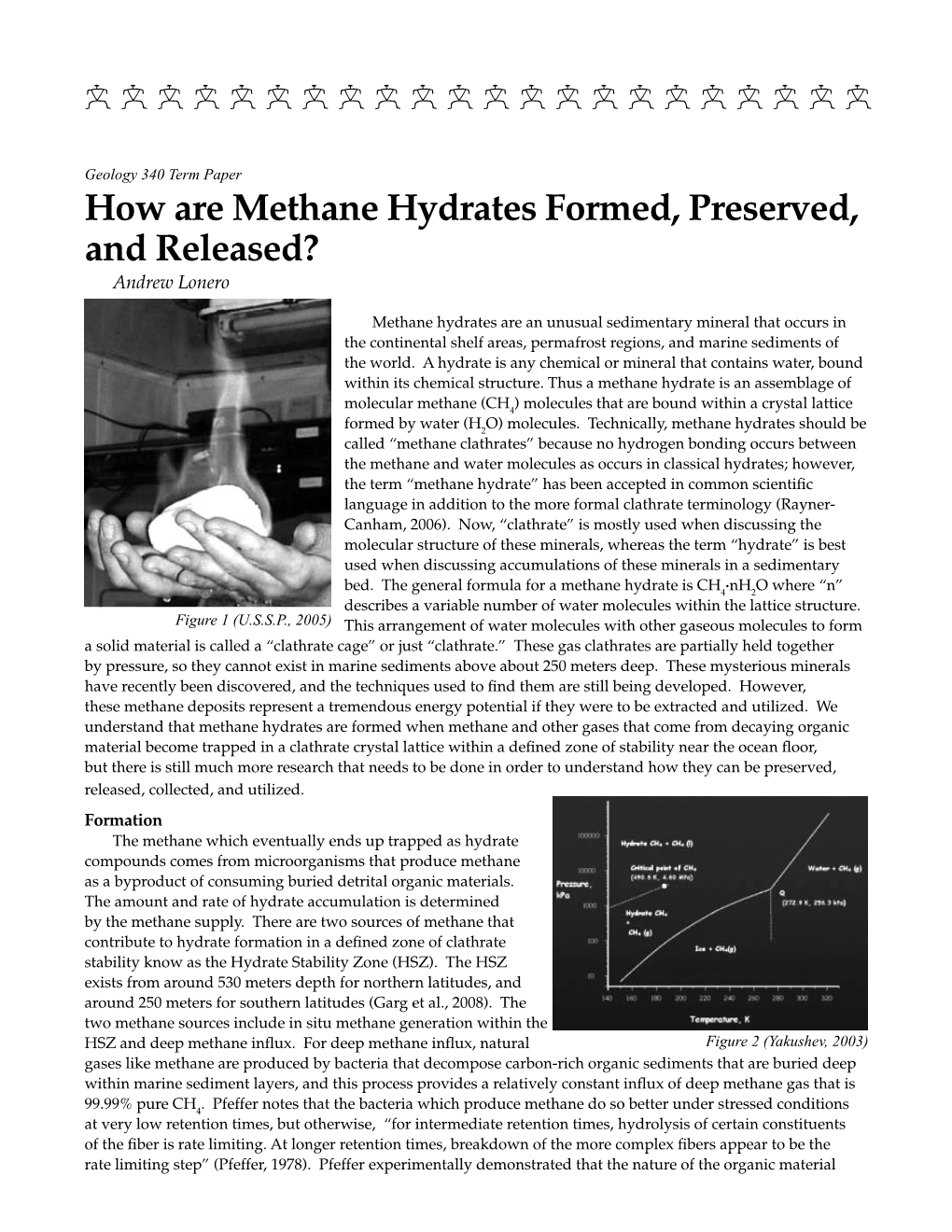
Load more
Recommended publications
-
Minimal Geological Methane Emissions During the Younger Dryas-Preboreal Abrupt Warming Event
UC San Diego UC San Diego Previously Published Works Title Minimal geological methane emissions during the Younger Dryas-Preboreal abrupt warming event. Permalink https://escholarship.org/uc/item/1j0249ms Journal Nature, 548(7668) ISSN 0028-0836 Authors Petrenko, Vasilii V Smith, Andrew M Schaefer, Hinrich et al. Publication Date 2017-08-01 DOI 10.1038/nature23316 Peer reviewed eScholarship.org Powered by the California Digital Library University of California LETTER doi:10.1038/nature23316 Minimal geological methane emissions during the Younger Dryas–Preboreal abrupt warming event Vasilii V. Petrenko1, Andrew M. Smith2, Hinrich Schaefer3, Katja Riedel3, Edward Brook4, Daniel Baggenstos5,6, Christina Harth5, Quan Hua2, Christo Buizert4, Adrian Schilt4, Xavier Fain7, Logan Mitchell4,8, Thomas Bauska4,9, Anais Orsi5,10, Ray F. Weiss5 & Jeffrey P. Severinghaus5 Methane (CH4) is a powerful greenhouse gas and plays a key part atmosphere can only produce combined estimates of natural geological in global atmospheric chemistry. Natural geological emissions and anthropogenic fossil CH4 emissions (refs 2, 12). (fossil methane vented naturally from marine and terrestrial Polar ice contains samples of the preindustrial atmosphere and seeps and mud volcanoes) are thought to contribute around offers the opportunity to quantify geological CH4 in the absence of 52 teragrams of methane per year to the global methane source, anthropogenic fossil CH4. A recent study used a combination of revised 13 13 about 10 per cent of the total, but both bottom-up methods source δ C isotopic signatures and published ice core δ CH4 data to 1 −1 2 (measuring emissions) and top-down approaches (measuring estimate natural geological CH4 at 51 ± 20 Tg CH4 yr (1σ range) , atmospheric mole fractions and isotopes)2 for constraining these in agreement with the bottom-up assessment of ref. -
Thermal Regeneration of Sulfuric Acid Hydrates After Irradiation ⇑ Mark J
Icarus 219 (2012) 561–566 Contents lists available at SciVerse ScienceDirect Icarus journal homepage: www.elsevier.com/locate/icarus Thermal regeneration of sulfuric acid hydrates after irradiation ⇑ Mark J. Loeffler , Reggie L. Hudson Astrochemistry Laboratory, NASA Goddard Space Flight Center, Mail Code 691, Greenbelt, MD 20771, United States article info abstract Article history: In an attempt to more completely understand the surface chemistry of the jovian icy satellites, we have Received 17 January 2012 investigated the effect of heating on two irradiated crystalline sulfuric acid hydrates, H2SO4Á4H2O and Revised 13 March 2012 H2SO4ÁH2O. At temperatures relevant to Europa and the warmer jovian satellites, post-irradiation heating Accepted 25 March 2012 recrystallized the amorphized samples and increased the intensities of the remaining hydrate’s infrared Available online 10 April 2012 absorptions. This thermal regeneration of the original hydrates was nearly 100% efficient, indicating that over geological times, thermally-induced phase transitions enhanced by temperature fluctuations will Keywords: reform a large fraction of crystalline hydrated sulfuric acid that is destroyed by radiation processing. Europa The work described is the first demonstration of the competition between radiation-induced amorphiza- Ices, IR spectroscopy Jupiter, Satellites tion and thermally-induced recrystallization in icy ionic solids relevant to the outer Solar System. Impact processes Published by Elsevier Inc. Cosmic rays 1. Introduction ic, salty, or acidic, could be transported to a surface by a variety of mechanisms (Kargel et al., 2000; Greenburg, 2010). Primordial sub- Remote sensing of Jupiter’s icy satellites has revealed that even surface SO2 (Noll et al., 1995) and CO2 (Moore et al., 1999) could though their surfaces are composed mostly of water ice (Kuiper, also be carried upward by geological processes. -
Toxicological Review of Chloral Hydrate (CAS No. 302-17-0) (PDF)
EPA/635/R-00/006 TOXICOLOGICAL REVIEW OF CHLORAL HYDRATE (CAS No. 302-17-0) In Support of Summary Information on the Integrated Risk Information System (IRIS) August 2000 U.S. Environmental Protection Agency Washington, DC DISCLAIMER This document has been reviewed in accordance with U.S. Environmental Protection Agency policy and approved for publication. Mention of trade names or commercial products does not constitute endorsement or recommendation for use. Note: This document may undergo revisions in the future. The most up-to-date version will be made available electronically via the IRIS Home Page at http://www.epa.gov/iris. ii CONTENTS—TOXICOLOGICAL REVIEW for CHLORAL HYDRATE (CAS No. 302-17-0) FOREWORD .................................................................v AUTHORS, CONTRIBUTORS, AND REVIEWERS ................................ vi 1. INTRODUCTION ..........................................................1 2. CHEMICAL AND PHYSICAL INFORMATION RELEVANT TO ASSESSMENTS ..... 2 3. TOXICOKINETICS RELEVANT TO ASSESSMENTS ............................3 4. HAZARD IDENTIFICATION ................................................6 4.1. STUDIES IN HUMANS - EPIDEMIOLOGY AND CASE REPORTS .................................................6 4.2. PRECHRONIC AND CHRONIC STUDIES AND CANCER BIOASSAYS IN ANIMALS ................................8 4.2.1. Oral ..........................................................8 4.2.2. Inhalation .....................................................12 4.3. REPRODUCTIVE/DEVELOPMENTAL STUDIES ..........................13 -
Greenhouse Carbon Balance of Wetlands: Methane Emission Versus Carbon Sequestration
T ellus (2001), 53B, 521–528 Copyright © Munksgaard, 2001 Printed in UK. All rights reserved TELLUS ISSN 0280–6509 Greenhouse carbon balance of wetlands: methane emission versus carbon sequestration By GARY J. WHITING1* and JEFFREY P. CHANTON2, 1Department of Biology, Chemistry, and Environmental Science, Christopher Newport University, Newport News, V irginia 23606, USA; 2Department of Oceanography, Florida State University, T allahassee, Florida 32306, USA (Manuscript received 1 August 2000; in final form 18 April 2001) ABSTRACT Carbon fixation under wetland anaerobic soil conditions provides unique conditions for long- term storage of carbon into histosols. However, this carbon sequestration process is intimately linked to methane emission from wetlands. The potential contribution of this emitted methane ff to the greenhouse e ect can be mitigated by the removal of atmospheric CO2 and storage into peat. The balance of CH4 and CO2 exchange can provide an index of a wetland’s greenhouse gas (carbon) contribution to the atmosphere. Here, we relate the atmospheric global warming potential of methane (GWPM) with annual methane emission/carbon dioxide exchange ratio of wetlands ranging from the boreal zone to the near-subtropics. This relationship permits one to determine the greenhouse carbon balance of wetlands by their contribution to or attenuation ff of the greenhouse e ect via CH4 emission or CO2 sink, respectively. We report annual measure- ments of the relationship between methane emission and net carbon fixation in three wetland ecosystems. The ratio of methane released to annual net carbon fixed varies from 0.05 to 0.20 on a molar basis. Although these wetlands function as a sink for CO2, the 21.8-fold greater infrared absorptivity of CH4 relative to CO2 (GWPM) over a relatively short time horizon (20 years) would indicate that the release of methane still contributes to the overall greenhouse ff e ect. -
The Interplay Between Sources of Methane And
THE INTERPLAY BETWEEN SOURCES OF METHANE AND BIOGENIC VOCS IN GLACIAL-INTERGLACIAL FLUCTUATIONS IN ATMOSPHERIC GREENHOUSE GAS CONCENTRATIONS AND THE GLOBAL CARBON CYCLE Jed O. Kaplan1, Gerd Folberth2, and Didier A. Hauglustaine3 1Institute of Plant Sciences, University of Bern, Bern, Switzerland; [email protected] 2School of Earth and Ocean Sciences, University of Victoria, Victoria BC, Canada; [email protected] 3Laboratoire des Sciences du Climat et de l’Environnement, Gif-sur-Yvette, France ; [email protected] ABSTRACT Recent analyses of ice core methane concentrations have suggested that methane emissions from wetlands were the primary driver for prehistoric changes in atmospheric methane. However, these data conflict as to the location of wetlands, magnitude of emissions, and the environmental controls on methane oxidation. The flux of other reactive trace gases to the atmosphere also controls apparent atmospheric methane concentrations because these compounds compete for the hydroxyl radical (OH), which is the primary atmospheric sink for methane. In a series of coupled biosphere-atmosphere chemistry-climate modelling experiments, we simulate the methane and biogenic volatile organic compound emissions from the terrestrial biosphere from the Last Glacial Maximum (LGM) to present. Using an atmospheric chemistry-climate model, we simulate the atmospheric concentrations of methane, the hydroxyl radical, and numerous other reactive trace gas species. Over the past 21,000 years methane emissions from wetlands increased slightly to the end of the Pleistocene, but then decreased again, reaching levels at the preindustrial Holocene that were similar to the LGM. Global wetland area decreased by 14% from LGM to preindustrial. However, emissions of biogenic volatile organic compounds (BVOCs) nearly doubled over the same period of time. -
Hydrate Risk Assessment and Restart-Procedure Optimization Of
Hydrate Risk Assessment and Restart- Procedure Optimization of an Offshore Well Using a Transient Hydrate Prediction Model L.E. Zerpa, E.D. Sloan, C.A. Koh, and A.K. Sum, Colorado School of Mines Summary This paper focuses on the application of the gas-hydrate model A produced-hydrocarbon stream from a wellhead encounters for- to study the hydrate-plugging risk of an offshore well with a typ- mation of solid gas-hydrate deposits, which plug flowlines and ical geometry and fluid properties from the Caratinga field located which are one of the most challenging problems in deep subsea fa- in the Campos basin, Brazil. Three different periods of the well cilities. This paper describes a gas-hydrate model for oil-dominated life are considered: an early stage with low production gas/oil ratio systems, which can be used for the design and optimization of facil- (GOR) and low water cut, a middle stage with an increased GOR, ities focusing on the prevention, management, and remediation of and a late stage with higher GOR and higher water cut. The hy- hydrates in flowlines. Using a typical geometry and fluid properties drate-plugging risk is estimated from the calculation of three per- of an offshore well from the Caratinga field located in the Campos formance measures (pressure drop along flowline, hydrate volume basin in Brazil, the gas-hydrate model is applied to study the hy- fraction in pipe, and hydrate-slurry relative viscosity) in an attempt drate-plugging risk at three different periods of the well life. Addi- to quantify the plugging risk. -
Synthesis of Methane Hydrate from Ice Powder Accelerated by Doping
www.nature.com/scientificreports OPEN Synthesis of Methane Hydrate from Ice Powder Accelerated by Doping Ethanol into Methane Gas Received: 2 May 2019 Yen-An Chen1, Liang-Kai Chu1, Che-Kang Chu1, Ryo Ohmura2 & Li-Jen Chen 1,2 Accepted: 13 August 2019 Clathrate hydrate is considered to be a potential medium for gas storage and transportation. Slow Published: xx xx xxxx kinetics of hydrate formation is a hindrance to the commercialized process development of such applications. The kinetics of methane hydrate formation from the reaction of ice powder and methane gas doped with/without saturated ethanol vapor at constant pressure of 16.55 ± 0.20 MPa and constant temperature ranging from −15 to −1.0 °C were investigated. The methane hydrate formation can be dramatically accelerated by simply doping ethanol into methane gas with ultralow ethanol concentration (<94 ppm by mole fraction) in the gas phase. For ethanol-doped system 80.1% of ice powder were converted into methane hydrate after a reaction time of 4 h, while only 26.6% of ice powder was converted into methane hydrate after a reaction time of 24 h when pure methane gas was used. Furthermore, this trace amount of ethanol could also substantially suppress the self-preservation efect to enhance the dissociation rate of methane hydrate (operated at 1 atm and temperatures below the ice melting point). In other words, a trace amount of ethanol doped in methane gas can act as a kinetic promoter for both the methane hydrate formation and dissociation. Clathrate hydrates are ice-like nonstoichiometric crystalline compounds formed by guest molecules encapsulated in the hydrogen bonded water cages at elevated pressures and low temperatures1. -
Methane Hydrate Stability and Anthropogenic Climate Change
Biogeosciences, 4, 521–544, 2007 www.biogeosciences.net/4/521/2007/ Biogeosciences © Author(s) 2007. This work is licensed under a Creative Commons License. Methane hydrate stability and anthropogenic climate change D. Archer University of Chicago, Department of the Geophysical Sciences, USA Received: 20 March 2007 – Published in Biogeosciences Discuss.: 3 April 2007 Revised: 14 June 2007 – Accepted: 19 July 2007 – Published: 25 July 2007 Abstract. Methane frozen into hydrate makes up a large 1 Methane in the carbon cycle reservoir of potentially volatile carbon below the sea floor and associated with permafrost soils. This reservoir intu- 1.1 Sources of methane itively seems precarious, because hydrate ice floats in water, and melts at Earth surface conditions. The hydrate reservoir 1.1.1 Juvenile methane is so large that if 10% of the methane were released to the at- Methane, CH , is the most chemically reduced form of car- mosphere within a few years, it would have an impact on the 4 bon. In the atmosphere and in parts of the biosphere con- Earth’s radiation budget equivalent to a factor of 10 increase trolled by the atmosphere, oxidized forms of carbon, such as in atmospheric CO . 2 CO , the carbonate ions in seawater, and CaCO , are most Hydrates are releasing methane to the atmosphere today in 2 3 stable. Methane is therefore a transient species in our at- response to anthropogenic warming, for example along the mosphere; its concentration must be maintained by ongoing Arctic coastline of Siberia. However most of the hydrates release. One source of methane to the atmosphere is the re- are located at depths in soils and ocean sediments where an- duced interior of the Earth, via volcanic gases and hydrother- thropogenic warming and any possible methane release will mal vents. -
Information on Selected Climate and Climate-Change Issues
INFORMATION ON SELECTED CLIMATE AND CLIMATE-CHANGE ISSUES By Harry F. Lins, Eric T. Sundquist, and Thomas A. Ager U.S. GEOLOGICAL SURVEY Open-File Report 88-718 Reston, Virginia 1988 DEPARTMENT OF THE INTERIOR DONALD PAUL MODEL, Secretary U.S. GEOLOGICAL SURVEY Dallas L. Peck, Director For additional information Copies of this report can be write to: purchased from: Office of the Director U.S. Geological Survey U.S. Geological Survey Books and Open-File Reports Section Reston, Virginia 22092 Box 25425 Federal Center, Bldg. 810 Denver, Colorado 80225 PREFACE During the spring and summer of 1988, large parts of the Nation were severely affected by intense heat and drought. In many areas agricultural productivity was significantly reduced. These events stimulated widespread concern not only for the immediate effects of severe drought, but also for the consequences of potential climatic change during the coming decades. Congress held hearings regarding these issues, and various agencies within the Executive Branch of government began preparing plans for dealing with the drought and potential climatic change. As part of the fact-finding process, the Assistant Secretary of the Interior for Water and Science asked the Geological Survey to prepare a briefing that would include basic information on climate, weather patterns, and drought; the greenhouse effect and global warming; and climatic change. The briefing was later updated and presented to the Secretary of the Interior. The Secretary then requested the Geological Survey to organize the briefing material in text form. The material contained in this report represents the Geological Survey response to the Secretary's request. -
Regenerative Development, the Way Forward to Saving Our Civilization. Dr
Regenerative development, the way forward to saving our civilization. Dr. Eduard Müller1 Abstract: Humankind has taken a dangerous path with its current global development trends. We are in a time of rapid population growth coupled with overconsumption and massive destruction of Nature. These trends, potentiated by technological developments have resulted in human induced impacts even in the most remote places. Change is accelerating and increasing in magnitude and consequences making it impossible to be in denial. Communication has never been better, faster and cheaper. We are flooded with alarming news from around the world yet we are continuing to promote degenerative development. In order to reverse the damage and allow for life on the planet to continue – though with changes – we need to implement regenerative development, based on a holistic approach that integrates six layers: 1) regeneration of functional landscapes, where we produce and conserve, maximizing ecosystem function; 2) social strengthening by community organization and development, to cope with adaptation to climate change and reduce sumptuous consumption patterns; 3) a new paradigm for economic development where people matter more than markets and money, measured according to the well-being of humans and all life forms; 4) conservation and valuation of living culture which is the necessary bond for community life, where local knowledge, values and traditions are shared within family, friends and the community as a whole, giving meaning to these terms; 5) rethinking and redesigning current political structures so they reflect true participatory democracy without the influence of money and power and especially fostering long term vision and actions that seek increased livelihoods and happiness and not only gross income, and most importantly; 6) fostering deep spiritual and value structures based on ethics, transparency and global well-being to allow humanity to live in peace with itself and Mother Earth. -
Methanol—Inhibitor Or Promoter of the Formation of Gas Hydrates from Deuterated Ice?
BOBEV AND TAIT: METHANOL—INHIBITOR OR PROMOTER? 1209 American Mineralogist, Volume 89, pages 1208–1214, 2004 Methanol—inhibitor or promoter of the formation of gas hydrates from deuterated ice? SVILEN BOBEV* AND KIMBERLY T. TAIT Manuel Lujan Jr. Neutron Scattering Center, LANSCE 12 MS H805, Los Alamos National Laboratory, Los Alamos, New Mexico 87545, U.S.A. ABSTRACT Kinetic studies are reported of the effect of methanol on the rate of formation of CO2- and CH4-hy- drates by means of in situ time-of-flight neutron powder diffraction. The experiments were carried out at temperatures ranging from 200 to 250 K and pressures up to 7 MPa. The samples were prepared from mixtures of ground, deuterated ice and deuterated methanol (up to 20 vol%), which were transformed in situ into CO2- or CH4-hydrates by pressurizing the systems with the corresponding gas. The observed rates of formation of hydrates are orders of magnitude higher than the rate of formation from pure deuterated ice under the same pressure and temperature conditions. Glycols and alcohols, methanol in particular, are long known as thermodynamic inhibitors of hydrate formation. Our study indicates that methanol can also act as a kinetic promoter for the formation of gas hydrates. Preliminary data suggest that the kinetics also depend strongly on concentration and the isotopic composition. INTRODUCTION particular, time-dependent neutron diffraction at a variety of tem- The crystal structures, thermodynamic models, and engineer- peratures and pressures has been effectively used to research the ing applications of CO2- and CH4-hydrates have been extensively kinetics of gas hydrate formation and dissociation (Henning et al. -
Wetlands, Carbon and Climate Change
Wetlands, Carbon and Climate Change William J. Mitsch Everglades Wetland Research Park, Florida Gulf Coast University, Naples Florida with collaboration of: Blanca Bernal, Amanda M. Nahlik, Ulo Mander, Li Zhang, Christopher Anderson, Sven E. Jørgensen, and Hans Brix Florida Gulf Coast University (USA), U.S. EPA, Tartu University (Estonia), Auburn University (USA), Copenhagen University (Denmark), and Aarhus University (Denmark) Old Global Carbon Budget with Wetlands Featured Pools: Pg (=1015 g) Fluxes: Pg/yr Source:Mitsch and Gosselink, 2007 Wetlands offer one of the best natural environments for sequestration and long-term storage of carbon…. …… and yet are also natural sources of greenhouse gases (GHG) to the atmosphere. Both of these processes are due to the same anaerobic condition caused by shallow water and saturated soils that are features of wetlands. Bloom et al./ Science (10 January 2010) suggested that wetlands and rice paddies contribute 227 Tg of CH4 and that 52 to 58% of methane emissions come from the tropics. They furthermore conclude that an increase in methane seen from 2003 to 2007 was due primarily due to warming in Arctic and mid-latitudes over that time. Bloom et al. 2010 Science 327: 322 Comparison of methane emissions and carbon sequestration in 18 wetlands around the world 140 120 y = 0.1418x + 11.855 Methane R² = 0.497 emissions, 100 g-C m-2 yr-1 80 60 40 20 0 -100 0 100 200 300 400 500 600 Carbon sequestration, g-C m-2 yr-1 • On average, methane emitted from wetlands, as carbon, is 14% of the wetland’s carbon sequestration.